J. Cent. South Univ. (2012) 19: 63-70
DOI: 10.1007/s11771-012-0973-x
UV-curing of hyperbranched polyurethane acrylate-polyurethane diacrylate/SiO2 dispersion and TGA/FTIR study of cured films
GAO Qiong-zhi(高琼芝)1, 2, LI Hong-qiang(李红强)1, ZENG Xing-rong(曾幸荣)1
1. College of Materials Science and Engineering, South China University of Technology, Guangzhou 510640, China;
2. College of Science, South China Agricultural University, Guangzhou 510640, China
? Central South University Press and Springer-Verlag Berlin Heidelberg 2012
Abstract: UV-curable hyperbranched polyurethane acrylate-polyurethane diacrylate/SiO2 dispersion (HBPUA-PUDA/SiO2) was prepared with isophorone diisocyanate (IPDI), hyperbranched polyester Boltorn H20 (H20), hydroxy-ethyl acrylate (HEA), polyethyleneglycol (PEG-200) and nano-SiO2. The UV curing kinetics of the films was investigated by FTIR. The results show that the curing speed of the films increases with the adding of nano-SiO2 and decreases with the adding of PUDA due to the slower chain movement. The thermal stability of the HBPUA-PUDA/SiO2 films was studied by using thermogravimetric analysis coupled with Fourier transform infrared spectroscopy (TGA/FTIR). The results show that all films exhibit two degradation stages located at about 320 and 440 °C corresponding to the degradation for hard segments of urethane-acrylate and the degradation for soft segment and polyester core. In addition, the results from the analysis of TGA/FTIR also indicate that the decomposition temperature of HBPUA-PUDA/SiO2 film is 15 °C higher than that obtained for pure polymer. The degradation mechanism was proposed according to TGA/FTIR results.
Key words: UV-curing; hyperbranched polyurethane acrylate; urethane diacrylate; TGA/FTIR; thermal degradation
1 Introduction
UV-curable coatings are becoming increasingly attractive for fast curing speed, lower energy consumption, VOC minimization and high quality products [1-3]. They have been used in furniture coatings and many industrial fields including elecotronics, printing and automotive. However, during the process of factual application, in order to obtain the desired properties of a coating, the prepolymers with higher viscosity are usually used in a formulation, which requires the use of reactive diluents to keep the formulation at an acceptable viscosity for application [4]. Meanwhile, it is now significant for chemical and coating industries to produce environmentally friendly products, therefore there is a general trend to reduce the content of reactive diluents and develop UV-curable formulations whose disadvantages can be eliminated.
It is difficult to achieve both rapid curing speed and lower viscosity for an oligomer by a conventional technique. At present, hyperbranched polymers provide an effective solution in this field because of their multiplicity of end groups and highly branched architecture, and exhibit properties different from those of linear counterparts [5-8]. One potential application for these polymers is used as UV-curable oligomer in coatings. Although progress is made in research of hyperbranched polymers for UV-curable coating systems [9-12], there are few reports on the thermal stability of hyperbranched UV-curable coatings.
The hyperbranched aliphatic polyester Boltorn TM H20 (H20) is the second generation of dendritic polyester having an average molecular mass of 1 747 g/mol and an average of 16 hydroxyl end groups per molecule. The functional groups located at the ends of polymer chain can be easily modified to obtain the UV-curable acrylate functionality.
In this work, Boltorn TM H20 and SiO2 were respectively introduced into UV-curable coatings to reduce viscosity, increase wear resistance and heat stability, which are beneficial for process of coating and improvement of combination properties. A new method for preparation of UV-curable HBPUA-PUDA/SiO2 dispersion is presented. The curing speed of HBPUA-PUDA/SiO2 dispersion is evaluated via Fourier transition infrared spectroscopy (FTIR). The thermal stability of HBPUA-PUDA/SiO2 films is studied by TGA/FTIR and the probable degradation mechanism is proposed according to TGA/FTIR results.
2 Experimental
2.1 Materials and reagents
Hyperbranched aliphatic polyester, Boltorn H20, was purchased from Perstorp AB, Perstorp, Sweden. Isophorone diisocyanate (IPDI) was supplied by BASF Co., Germany. Hydroxyethyl acrylate (HEA) was purchased from Shanghai Runjie Chemical Reagent Factory, China. Polyethylene glycol (PEG-200), tetraethylorthosilicate (TEOS), absolute ethyl alcohol, hydrochloric acid (HCl) and tetrahydrofuran (THF) were all purchased from Tianjin No. 1 Chemical Reagent Factory, China. 3-glycidoxypropyltrimethoxysilane (A-174) and dibutyltin dilaurate (DBTDL) were purchased from GE. Co., USA. 2-hydroxyl-2-methyl- l-phenyl-1-propanone (Darocur 1173), as a photoinitiator, was supplied by Ciba Co., Switzerland. All chemicals were used as received without further purification.
2.2 Synthesis of HBPUA-PUDA dispersion
The HBPUA-PUDA dispersion was synthesized via a two-step procedure. First, urethane monoacrylate (IPDI/HEA) was obtained by a reaction between the secondary cycloaliphatic NCO group (NCOsec) of IPDI and the OH group of HEA with DBTDL as catalyst. IPDI (22.22 g, 0.10 mol), DBTDL (0.11 g) and hydroquinone (0.07 g) were mixed in a four-neck boiling flask (250 mL) equipped with a mechanical stirrer, a reflux condenser, a thermometer and with a N2 inlet. Then, HEA (11.61 g, 0.10 mol) was slowly dropped into the mixture in 40 min. The mixture was stirred at 35 °C and the reaction continued until the content of isocyanate reached the theoretical value of mono-isocyanate (IPDI/HEA) by titrations. Secondly, the resultant IPDI/HEA reacted with H20 and PEG-200 to get HBPUA-PUDA dispersion. IPDI/HEA was reacted with H20 (7.65 g, 0.004 4 mol) and PEG (3.00 g, 0.015mol) dissolved in THF at 70 °C. The reaction continued until the content of NCO group was less than 0.5% (mass fraction) by titrations. Clear liquid of hyperbranched polyurethane acrylate- polyurethane diacrylate (HBPUA-PUDA) dispersion was obtained after the THF was evaporated completely. In the second step of reaction, if the resultant IPDI/HEA reacted with H20 (10.94 g, 0.006 25 mol) dissolved in THF at 70 °C for 3.5 h, then HBPUA oligomer would be obtained. The synthesis process is shown in Scheme 1.
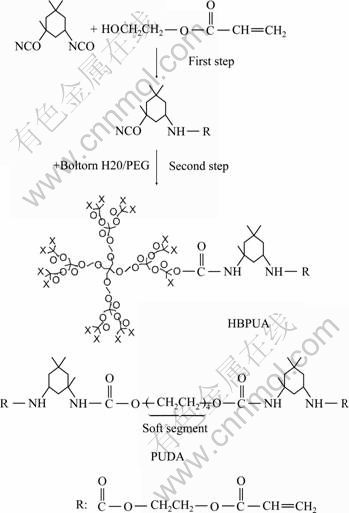
Scheme 1 Synthesis of HBPUA-PUDA dispersion
2.3 Preparation of organic nano-SiO2
TEOS (0.1 mol) and absolute ethyl alcohol (0.5 mol) were mixed in a conical flask (250 mL) equipped with a magnetic stirrer and a dropping funnel at room temperature. Then, the HCl aqueous solution (0.03 mol HCl dissolved in 0.3 mol H2O) in the dropping funnel was added by dropwise into the conical flask under magnetic stirring within 15 min; subsequently, A-174 (0.033 mol) was added by dropwise into the conical flask under magnetic stirring within 15 min. Then, organic modified nano-SiO2 was obtained [13]. The synthesis process is shown in Scheme 2.
2.4 Preparation of HBPUA-PUDA/SiO2 film
HBPUA-PUDA dispersion and organic nano-SiO2 (25%, mass fraction) were firstly mixed with 2% (mass fraction) Darocur 1173 (based on the solid content) and stirred until the dispersion was homogeneous, and then the mixture was coated on glass plate or tin plate by an applicator with a 100 μm gap and cured to form HBPUA-PUDA/SiO2 IPN film by exposing to a UV lamp (365 nm, 2 kW, 80 W/cm).
2.5 Characterizations
Fourier transform infrared (FTIR) spectroscopy was recorded in the spectral range of 4 000-500 cm-1 with a 4 cm-1 resolution and 16 scans on a VECTOR 33 FTIR spectrometer (Bruker, Germany), and the samples were smeared on a KBr wafer to form a thin film.
TGA/FTIR measurements were carried out on a TGA Pyris 1 coupled with a Spectrum GX FTIR system (Perkin Elmer). Samples with the mass ranging from 15 to 20 mg were heated from 50 to 600 °C at a rate of 10 °C/min under a dry nitrogen atmosphere. The flow rate of nitrogen into the cell for TGA/FTIR experiments was 60 mL/min. Both the FTIR cell and the heated line transferring evolved gases from the TGA to FTIR were maintained at 250 °C. FTIR spectra were recorded in the spectral range of 4 000-650 cm-1 with a 4 cm-1 resolution and 8 scans.
3 Results and discussion
3.1 FTIR spectra of HBPUA-PUDA dispersion and IPDI/HEA
Figure 1 shows the FTIR spectra of IPDI/HEA and HBPUA-PUDA dispersion. In Fig. 1, NCO stretching absorption appears at 2 270 cm-1 for IPDI/HEA and there is no absorption peak for HBPUA-PUDA. The spectra in HBPUA-PUDA show the absorption peaks of typical polyurethane at 3 340-3 380 cm-1 (NH, hydrogen- bonded), 2 870-2 975 cm-1 (CH2 and CH3), 1 720 cm-1 (C=O) and 1 538 cm-1 (NH). In addition, the spectra also show the absorption peaks at 1 636 cm-1 (C=C), 1 409 cm-1 (=CH2) and 810 cm-1 (=CH), which confirms that C=C bonds in HEA have been incorporated into the polyurethane chains.
3.2 Effect of UV radiation time on conversion of C=C bond
The conversion ratio of C=C is calculated by the area of characteristic absorption peak of acrylic ester via FTIR according to the methods in Refs. [6] and [8], then the conversion of C=C is calculated based on the following equation:
(1)
where X is the conversion ratio of C=C; A810 and A1 720 are the calibration peak areas, at 810 cm–1 and 1 720 cm–1, respectively. (A810/A1 720)0 is the ratio of A810 to A1 720 before UV radiation, and (A810/A1 720)t is the ratio of A810 to A1 720 at different UV radiation time.
The effect of UV radiation time on the conversion of C=C bonds is evaluated by FT-IR. From Figs. 2-4, it is found that, in the initial stage of UV radiation, the conversion ratio of C=C increases fast with the increase of radiation time. The maximum value of conversion ratio of C=C of HBPUA oligomer is 81% which is the highest among the above three samples. This result is due to the lower viscosity of spherical structure of HBPUA [12]. The radiation time of HBPUA is 83 s, which is faster than HBPUA-PUDA dispersion, on account of the slower movement of longer chain in PUDA. Compared with the curing rate of HBPUA-PDUA dispersion, it is found that curing speed and conversion ratio of C=C bonds are improved slightly when organic modified nano-SiO2 is added to HBPUA-PUDA dispersion, which is attributed to organic modified nano-SiO2 with small size and quick movement served as cross-linking agent.
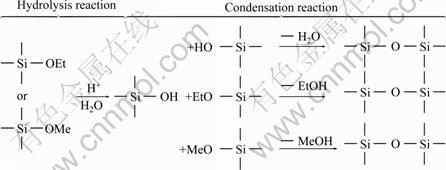
Scheme 2 Sol-gel reaction
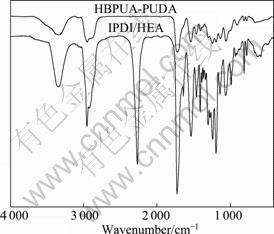
Fig. 1 FTIR spectra of IPDI/HEA and HBPUA-PUDA dispersion
3.3 Thermal analysis of UV-cured HBPUA-PUDA/ SiO2 films
3.3.1 TG
Figure 5 shows the TG and their corresponding derivative mass loss curves (DTG), respectively, for the films of HBPUA, HBPUA-PUDA and HBPUA-PUDA/ SiO2.
From Fig. 5, it can be seen that, all samples exhibit two degradation stages located at about 320 and 440 °C. The mass loss in the first and second stages is different. It is reported that the first mass loss during thermal degradation of HBPUA and PUDA is due to the degradation of the hard segment as a consequence of the relatively low thermal stability of the urethane-acrylate terminal groups. Whereas, the second mass loss is associated with soft segment or hyperbranched polyester core decomposition [14]. Furthermore, it is suggested that the amount of mass loss at each degradation stage might be used as a quantitative measurement of the hard terminal groups and soft or hyprebranched polyerster core content in samples. Thus, by using this approximation, the amount of the urethane-acrylate terminal groups in HBPUA and PUDA prepared in this work is about 49% (mass fraction), which is close to 56% (mass fraction) calculated by stoichiometry. This implies that the urethane-acrylate is less thermally-stable, and their degradation is depended on the isocyanate nature [15].
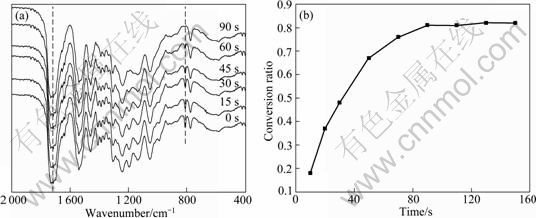
Fig. 2 UV spectra (a) and effect of UV radiation time on conversion ratio of C=C bond (b) in UV-curable HBPUA oligomer
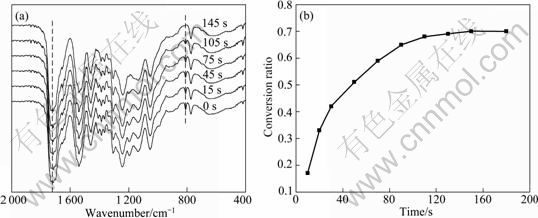
Fig. 3 UV spectra (a) and effect of UV radiation time on conversion ratio of C=C bond (b) in UV-curable HBPUA-PUDA dispersion
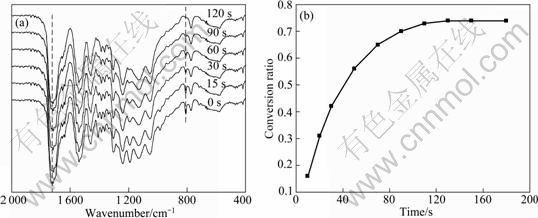
Fig. 4 UV spectra (a) and effect of UV radiation time on conversion ratio of C=C bond (b) in UV-curable HBPUA-PUDA/SiO2 dispersion
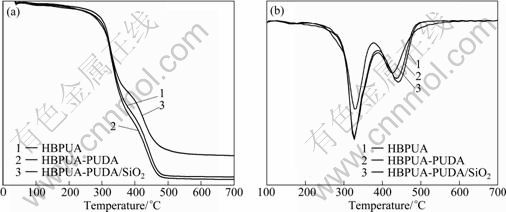
Fig.5 TG curves (a) and DTG curves (b) of films
Form Fig. 5, it is generally believed that the introduction of SiO2 into HBPUA-PUDA could improve its thermal stability because the dispersed SiO2 in the HBPUA-PUDA/SiO2 film hinders the permeability of volatile degradation products out of the materials, i.e. the inorganic material generates a “barrier effect”, which delays the release of thermal degradation products in comparison with the virgin polymer [16-17]. In this case, the increase in decomposition temperature of the first stage for SiO2 is about 15 °C higher than that obtained for pure polymer. The residue is about 20% (mass fraction) of SiO2 which is close to the content of HBPUA-PUDA/SiO2 film.
3.3.2 TGA/FTIR
In order to investigate the thermal stability and degradation mechanism of UV-cured films, TGA coupled FTIR measurement was carried out. The results of TGA/FTIR analysis are shown in Figs. 6, 7 and 8, respectively.
Stack plots of FTIR spectra obtained during thermal decomposition of HBPUA and HBPUA-PUDA films without SiO2 are shown in Figs. 6 and 7. As it could be noticed, spectrum obtained during the first gas emission shows some difference from that obtained in the second stage. The former shows mainly bands at 2 320 cm-1 (attributed to carbon dioxide and —NCO group), and peaks at 2 918 cm-1 (related to C—H stretching vibrations), indicating that the most of evolved gases at this stage possess hydrocarbon fragments (probably derived from cyclohexyl group) and CO2. Based on the mechanism proposed by PETROVIC et al [18] for thermal decomposition of segmented polyurethanes, it is reasonable to assume that the presence of CO2 is due to the decomposition of urethane groups according to mechanism displayed in Scheme 3, while the presence of C—H stretching vibrations is related to methylene groups from both cyclohexyl and polyethleneglycol. Spectra also show a small band at 1 089 cm-1, which can be attributed to C—O stretching vibration of an ether group and another small broad band in 4 000-3 500 cm-1 range, which can be related to O—H stretching vibrations from either water or hydroxyl-terminated compounds, and N—H stretching vibrations from either urea or amine groups. In agreement with this observation, similar compounds are detected [14, 19-20] during thermal decomposition of polyurethanes.
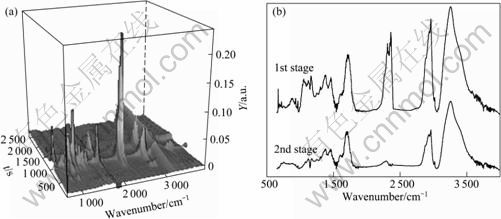
Fig. 6 Stacked plot of FTIR spectra (a) and FTIR spectra obtained at maximum evolution rate for each decomposition stage (b) of thermal degradation of HBPUA cured film
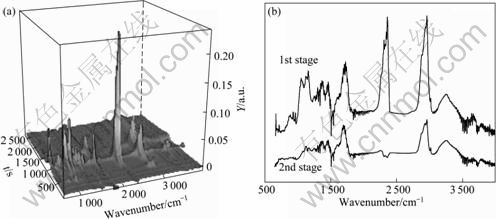
Fig. 7 Stacked plot of FTIR spectra (a) and FTIR spectra obtained at maximum evolution rate for each decomposition stage (b) of thermal degradation of HBPUA-PUDA cured film
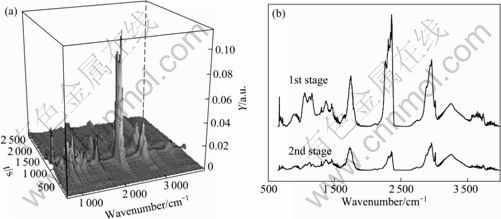
Fig. 8 Stacked plot of FTIR spectra (a) and FTIR spectra obtained at maximum evolution rate for each decomposition step (b) of thermal degradation of HBPUA-PUDA/SiO2 film
In contrast, the spectrum obtained during the second stage (except sample 3) shows no bands at 2 320 cm-1 (attributed to carbon dioxide and —NCO group), but displays the bands at 2 945 cm-1 which are related to C—H stretching vibrations for methylene and methyl groups from thermal degradation of HBPUA or HBPUA-PUDA, and the existence of these groups is confirmed by the presence of bands at 1 438 cm-1. A carbonyl band at 1 720 cm-1 related to C—O vibration of the ether group from HBPUA and HBPUA-PUDA is also observed. HERRERA et al [21] reported similar absorption bands during the second degradation stage of thermoplastic polyurethanes based on MDI. They claimed (with exception of carbonyl band) that the absorption bands obtained were typical absorptions of butyl ether. ORZESKO and KOLBRECKI [19] studied the thermal degradation of polyurethanes by using polyethylene glycol as model compound for soft segments and found aldehydes in the thermal decomposition products of this compound. So, the thermal degradation of HBPUA-PUDA can also generate aldehydes (possibly butanal) which can explain the carbonyl band at 1 720 cm-1.
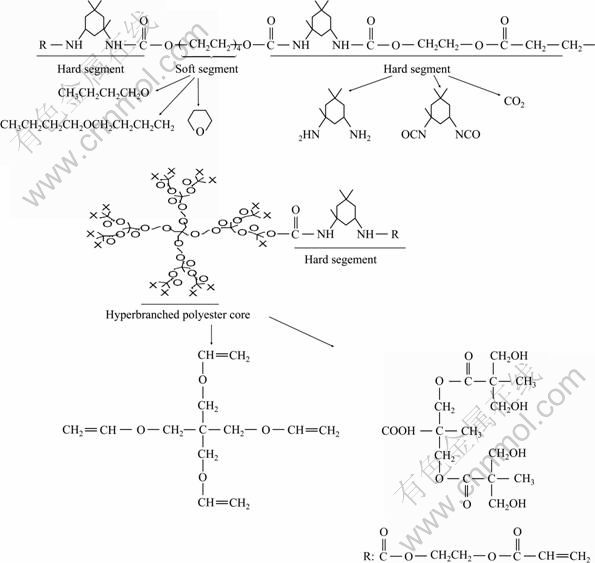
Scheme 3 Suggested mechanism for thermal degradation of HBPUA-PUDA/SiO2 films
From Fig. 8, it can be seen that, the film with SiO2 exhibits a different degradation path which includes the presence of CO2 in the second stage from the other two. Because the dispersed SiO2 in IPN hinders the permeability of volatile degradation products out of the materials, CO2 is delayed to release in the second stage which occurs in the first degradation stage for the virgin polymer [16-17].
4 Conclusions
UV-curable HBPUA-PUDA/SiO2 dispersion was prepared with IPDI, H20, HEA, PEG-200, and organic nano-SiO2. The results of UV curing kinetics of the HBPUA-PUDA/SiO2 films from FTIR analysis show that the curing speed of the films increases with the adding of nano-SiO2 and decreases with the adding of PUDA because of its slower chain movement. The results of TGA/FTIR analysis show that the thermal degradation of both HBPUA-PUDA and HBPUA- PUDA/SiO2 films occurs in two stages located at about 320 and 440 °C, respectively, indicating that the hard segment of urethane-acrylate is less thermally-stable than the soft segment and hyperbranched polyester core. Furthermore, the thermal stability of the film with SiO2 is effectively improved, and the decomposition temperature of HBPUA-PUDA/SiO2 films is 15 °C higher than that of the pure polymer.
References
[1] NEBIOGLU A, SOUCEK M D. Optimization of UV curable acrylated polyester-polyurethane/polysiloxane ceramer coatings using a response surface methodology [J]. Coatings Technology and Research, 2006, 3(1): 61-68.
[2] HE Jian-yun, ZHOU Liang, SOUCEK M D. UV-curable hybrid coatings based on vinyl functionalized siloxane oligomer and acrylated polyester [J]. Journal of Applied Polymer Science, 2007, 105(4): 2376-2386.
[3] PICHAVANT L, COQUERET X. Optimization of UV-curable acrylate-based protective coating by experimental design [J]. Progress in Organic Coatings, 2008, 63: 55-62.
[4] DECKER C. Photoinitiated crosslinking polymerization [J]. Progress in Polymer Science, 1996, 21: 593-650.
[5] KIM Y H. Hyperbranched polymers 10 years after [J]. Journal of Polymer Science Part A: Polymer Chemistry, 1998, 36(11): 1685-1698.
[6] LIN De, KOU Hui-guang, SHI Wen-fang. Photopolymerizaton of hyperbranched aliphatic acrylated poly (amide ester). II. Photopolymerization kinetics [J]. Journal of Applied Polymer Science, 2001, 82(7): 1637-1641.
[7] ASIF A, HU Li-hua, SHI Wen-fang. Synthesis, rheological, and thermal properties of waterborne hyperbranched polyurethane acrylate dispersions for UV curable coatings [J]. Colloid Polymer Science, 2009, 287(9): 1041-1049.
[8] TASIC S D, BOZIC B, DUNJIC B. Synthesis of new hyperbranched urethane-acrylates and their evaluation in UV-curable coatings [J]. Progress in Organic Coatings, 2004, 51: 321-328
[9] KOU Hui-guang, ASIF A, SHI Wen-fang. Photopolymerizable acrylated hyperbranched polyisophthalesters used for photorefractive materials I. Synthesis and characterization [J]. European Polymer Journal, 2002, 38(10): 1931-1936.
[10] WANG Sheng-jie, FAN Xiao-dong, KONG Jie, LU Jian-ren. Synthesis, characterization and UV curing kinetics of hyperbranched polysiloxysilanes from A2 and CB2 type monomers [J]. Polymer, 2009, 50(15): 3587-3594.
[11] MISHRA S, MISHRA A K, RAJU K V S N. Synthesis and property study of UV-curable hyperbranched polyurethane acrylate/ZnO hybrid coatings [J]. European Polymer Journal, 2009, 45(3): 960-966.
[12] KUMARI S, MISHRA A K, CHATTOPADHYAY D K, RAJU K V S N. Synthesis and characterization of hyperbranched polyesters and polyurethane coatings [J]. Journal of Polymer Science Part A: Polymer Chemistry, 2007, 45(13): 2673-2688.
[13] LIN Jin-na, HOU You-jun, ZENG Xing-rong. The stability of organic silicone/SiO2 hybrid coating by sol-gel process [J]. Polymer Materials Science & Engineering, 2007, 33(2): 128-130. (in Chinese)
[14] SIEGLER M V S, BURROWS M. Thermal degradation of polyurethanes investigated by direct pyrolysis in the mass spectrometer [J]. Macromolecule Chemistry, 1980, 181(10): 2161-2173.
[15] COUTINHO F, DELPECH M, ALVES T, FERREIRA A. Degradation profiles of cast films of polyurethane and poly (urethane-urea) aqueous dispersions based on hydroxyl-terminated polibutadien and different diisocyanate [J]. Polymer Degradation and Stability, 2003, 81(1): 19-27.
[16] BERTA M, LINDSAY C, PANS G, CAMINO G. Effect of chemical structure on combustion and thermal behavior of polyurethane elastomer layered silicate nanocomposites [J]. Polymer Degradation and Stability, 2006, 91(5): 1179-1191.
[17] MOON S Y, KIM J K, NAH C, LEE Y S. Polyurethane/montmorillonite nanoorganoclay hybrid as chain extenders [J]. European Polymer Journal, 2004, 40(8): 1615-1621.
[18] PETROVC Z S, ZAVARGO Z, FLYN J H, MACKNIGHT W J. Thermal degradation of segmented polyurethanes [J]. Journal of Applied Polymer Science, 1994, 51(6): 1087-1095.
[19] ORZESKO A, KOLBRECKI A. Thermal degradation of polyurethanes. Model compounds [J]. Journal of Applied Polymer Science, 1980, 25: 2969-2973.
[20] GRASSIE N, ZULFIQAR M. Thermal degradation of the polyurethane from 1,4-butanediol and methylene bis (4-phenyl isocyanate) [J]. Journal of Polymer Science, Polymer Chemistry Ed, 1978, 16: 1563-1574.
[21] HERRERA M, MATUSCHEK G, KETTRUP A. Thermal degradation of thermoplastic polyurethanes elastomers (TPU) based on MDI [J]. Polymer Degradation and Stability, 2002, 78(2): 323-331.
(Edited by YANG Bing)
Received date: 2010-12-31; Accepted date: 2011-05-08
Corresponding author: ZENG Xing-rong, Professor, PhD; Tel: +86-20-87114248; E-mail: psxrzeng@gmail.com