Trans. Nonferrous Met. Soc. China 24(2014) s112-s118
Strategies to suppress grain growth of nanocrystalline aluminum
Gowoon JEONG, Jooyoung PARK, Singon KANG, Hyunjoo CHOI
School of Advanced Materials Engineering, Kookmin University, 77 Jeongneung-ro, Seongbuk-gu, Seoul, 136-702, Korea
Received 18 June 2013; accepted 9 November 2013
Abstract: The grain growth behaviors of nanocrystalline aluminum, alloy and composite are compared. First, nanocrystalline aluminum is fabricated by consolidation of ball-milled powder. Second, nanocrystalline aluminum alloy is designed to have elements such as Mn, Zr, and Misch metals, which can form thermally stable second phases at grain boundaries and also drag the movement of grain boundaries. Third, nanocrystalline aluminum-based composites containing multi-walled carbon nanotubes (MWCNTs) are also prepared because MWCNTs are expected to be located at grain boundaries and to suppress the grain growth of nanocrystalline aluminum. These three types of samples are annealed at 550 °C for up to 5 d and the effect of annealing time on Vickers hardness of the samples is compared. As a result, MWCNTs are found to be most effective to impede grain growth of nanocrystalline aluminum.
Key words: aluminum (Al); carbon nanotubes (CNTs); composites; nanocrystalline; grain growth
1 Introduction
Aluminum ranks second as the most frequently used metal after steel, possibly stemming from its economic saving and lightweight natures [1-3]. However, its inherit poor mechanical properties such as low specific stiffness and strength have restricted its usages as structural parts in a variety of industries.
Nano-structured materials, whose structural elements, clusters, crystallites or molecules, have dimensions ranging below 100 nm, have attracted a great deal of attention due to the remarkable progresses in fundamental mechanical properties [4-9]. Numerous studies have so far been conducted to investigate the effects of grain size on the mechanical behavior of aluminum as well as to develop nanocrystalline aluminum [10-22]. In this grain size regime, plastic deformation by means of intragranular slip becomes to lose its significance and hence nanocrystalline aluminum shows significantly different deformation behavior from that of ultrafine crystalline (grain sizes in the range from 100 to 500 nm) or microcrystalline (grain sizes larger than 500 nm) aluminum [10-18]. This implies that the superior strength, positively deviated from Hall-Petch relation, can be achieved as the grain size of aluminum is reduced down to tens of nanometers [18,19]. Hence, a number of techniques have so far been introduced to produce nanocrystalline aluminum, by means of top-down methods such as severe plastic deformation [20] and mechanical milling [18,19] as well as bottom-up methods including gas condensation [21] and crystallization of amorphous alloys [22].
Despite considerable progress in the field of nanocrystalline aluminum, the thermally unstable structure is one of major challenges to be overcome; the large volume fraction of grain boundaries comes with an inherent instability. Hence, grain growth commonly induces significant softening of metals even at elevated temperatures. With this scope, attention has currently shifted to the problem of stabilizing nanocrystalline structures. Researchers have recently reported that the proper alloying design may lower the kinetic energy for grain growth, providing thermodynamically stabilized grain boundaries [23-27]; the grain boundary energy can be lowered by the segregation of alloying elements. However, it has not been well attended for nanocrystalline aluminum.
In the present work, we employ two strategies to impede grain growth of nanocrystalline aluminum at high temperatures: 1) precipitation of thermally stable second phases at grain boundaries; 2) reinforcement of the aluminum matrix using thermally stable multi-walled carbon nanotubes (MWCNTs). Three types of samples including nanocrystalline aluminum, alloy and composite were annealed at 550 °C for up to 5 d and Vickers hardness was measured at every time step to indirectly compare the grain growth behaviors of the samples.
2 Experimental
2.1 Powder process
A ball-milling process via an attrition mill (KMC Co. Ltd., KMC-1BV, Korea) was utilized for 1) grain size refinement of aluminum, 2) mechanical alloying and 3) dispersion of multi-walled CNTs (MWCNTs) in aluminum powder, in order to finally produce three different powders of nanocrystalline aluminum, alloy and composite.
Nanocrystalline aluminum powder was fabricated by ball-milling of the starting aluminum powder for 24 h. Gas-atomized aluminum powder (150 μm, 99.5% in purity, Changsung Co. Ltd.) was charged in a stainless steel chamber with stainless steel balls of 5 mm in diameter at a mass ratio of 1:15. 1% (mass fraction) stearic acid (CH3(CH2)16COOH) was added as a process control agent to prevent excessive cold welding among powders. The powder and balls were stirred by horizontal impellers attached to a vertical shaft rotating at 500 r/min. The milling process was performed under argon atmosphere to prevent the oxidation of powder.
To fabricate the nanocrystalline Al-11.9%Cu- 3.5%Ce-0.3%Zr-0.1%Mn (mass fraction) alloy, all element powders were ball-milled together for 24 h under the same condition with nanocrystalline aluminum. Cerium (165 μm, 99.9% in purity, Sigma Aldrich Korea Co. Ltd.) and copper (180 μm, 99.9% in purity, High Purity Chemicals Co. Ltd.) are expected to form Al8CeCu4 precipitates to impede the grain growth of aluminum. Further, zirconium (700 μm, 99.5% in purity, Sigma Aldrich Korea Co. Ltd.) and manganese (75 μm, 99% in purity, Sigma Aldrich Korea Co. Ltd.) are added with a purpose of solid solution strengthening and further grain refinement.
Nanocrystalline aluminum matrix composite powder containing 7.5% (volume fraction) of MWCNTs was produced through two-step ball-milling processes: monolithic aluminum powder was solely ball-milled for 18 h; then the aluminum powder was further ball-milled with MWCNTs (~20 nm in diameter, and ~5 μm in length, supplied from Applied Carbon Nano Co. Ltd.) for 6 h; hence, the total milling time was kept to be 24 h. Milling was conducted under the same condition with nanocrystalline aluminum or alloy although, in the second step, the composite powder was ball-milled at a 600 r/min to induce sufficient energy on MWCNTs so that MWCNTs can be uniformly dispersed in aluminum powder.
The powder size is significantly reduced during the attrition milling process, resulting in difficulties in consolidation. Therefore, planetary milling was utilized to increase the powder size by cold welding of the attrition-milled powder. Stainless steel bowls (500 mL) were filled with the milled powder (50 g) and stainless steel balls (~5 mm in diameter, 750 g) without any process control agents. Planetary milling was performed with a rotation speed of 200 r/min for 1.5 h.
2.2 Sintering
To obtain fully dense specimens, hot rolling was utilized. Prior to hot rolling, stearic acid was removed by heat treatment of ball-milled powder at 500 °C for 30 min. Then, powder was packed in a one-end-sealed copper tube (outer diameter: 45 mm, length: 150 mm, and thickness: 1.2 mm), compacted with pressure of ~200 MPa at room temperature. Then, the other end of the copper tube was also sealed. The sample was heated to a predetermined temperature of 480 °C; it required ~40 min upon heating the sample. Rolling was conducted with every 12% reduction. After 19 passes, the sample thickness was reduced to be ~1.7 mm. The copper container was mechanically peeled off.
2.3 Microstructure observation
The morphology of powder was observed using scanning electron microscope (SEM, JEOL, JSM 2001F, Japan). Specimens were attached to a carbon tape for SEM analysis. The microstructure with a high magnification of as-rolled specimens was examined by high-resolution transmission electron microscopy (HRTEM, JEOL 2100FX). The operating voltage was 200 keV in JEOL 2100FX. Thin foil specimens from the composite sheets were carefully prepared by polishing and an ion beam milling method (Gatan, PIPS 691, Oxford, U.K.) by double-sided Ar+ ion-beam etching at acceleration voltage of 2.0-3.5 kV. The angle between the ion beam and the surface of specimens was set at approximately from 4° to 8°. The presence of MWCNTs was confirmed by Raman spectroscopy (LabRam Aramis, Horiba Jobin Yvon, France). The spectra were collected under the ambient conditions using the 514.5 nm line of an argon-ion laser.
2.4 Heat-treatment and grain size measurement
The grain growth behavior of three different samples were examined by heat-treatment of samples at 550 °C for up to 120 h. The grain size and the composition of the samples were analyzed using X-ray diffraction (XRD, Rigaku, CN2301) with a Cu Kα radiation source (λ=1.5405
). XRD patterns were collected typically over 20°-100° in 2θ. The XRD peak broadening was attributed to the refinement of grains. The (111) and (200) reflections of aluminum were used to estimate the average grain size through the Scherrer formula [28] as
(1)
where βg(2θ) is the breadth at half maximum intensity of the peak, λ is the wave length of the X-ray radiation, θ is the Bragg angle and d is the average grain size. Micro Vickers hardness tester (Mitutoyo, Japan, HM-211) was utilized to measure the hardness, according to the heat-treatment time, on specimens with an indenter load of 3 N. Prior to measurement, the specimens were mechanically polished using SiC papers (up to #2000).
3 Results and discussion
Figure 1 shows SEM images of Al-MWCNTs composite powders: Fig. 1(a) exhibits powder morphology before planetary milling, Fig. 1(b) shows its magnified image, Fig. 1(c) exhibits powder morphology after planetary milling, and Fig. 1(d) shows its magnified image. The composite powder is significantly deformed to be flat-shaped during attrition milling, as shown in Fig. 1(a). The average diameter of the powder is approximately a few hundred of nanometers. In the magnified image (Fig. 1(b)), MWCNTs seem to be located on the surface of powder rather than to be dispersed or embedded inside the powder. In this case, MWCNTs may impede the diffusion welding among powders during a consolidation process. The flat-shaped composite powder is planetary-milled and its shape becomes less flat as shown in Fig. 1(c). In contrast to the attrition-milled powders, few MWCNTs are observed on the surface of the powder (Fig. 1(d)), providing easier consolidation routes for composite powder.
There are two possibilities for reasons why MWCNTs are not observed on the surface of powder after planetary milling: the destruction of MWCNTs by significant mechanical energy induced during planetary milling, and the dispersion of MWCNTs inside soft aluminum powder. Figure 2 shows Raman spectra of initial MWCNTs, powder after milling and composite sheet, respectively. Al-MWCNTs composite sheet and powder exhibit two broad peaks at 1346 and 1593 cm-1, corresponding to the typical D band and G band of MWCNTs, respectively [29]. Therefore, it is highly probable that the MWCNTs are dispersed inside the aluminum powder during the planetary milling rather than are destroyed. As the composite powder is severely milled-peaks shift toward the higher wave number, possibly originating from the compressive force in MWCNTs by a high-velocity impact of balls on the powder [30]. Furthermore, a number of defects might be generated due to the severe ball-powder-ball collision, providing a higher intensity of D peak over G peak for the ball-milled specimens. Despite of the defect generation, Raman characterization confirms the retention of the initial structure of MWCNTs as well as the presence of MWCNTs even after the hot working process.
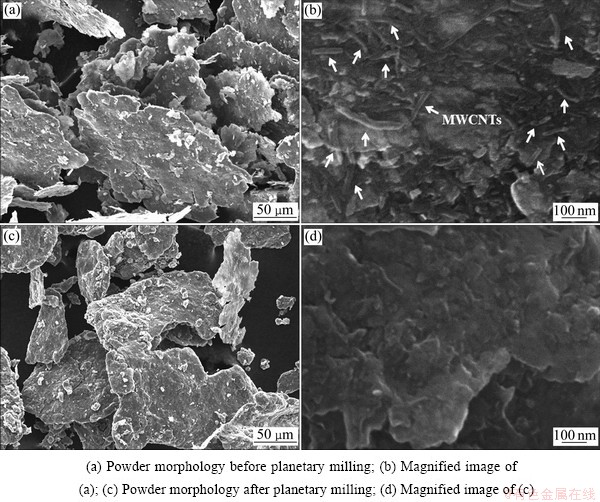
Fig. 1 SEM images of Al-MWCNTs composite powders
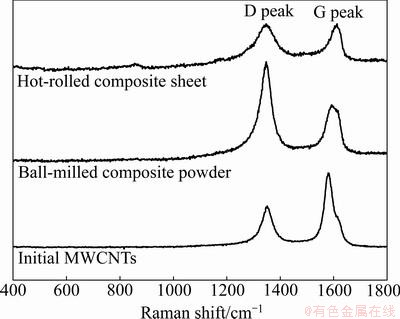
Fig. 2 Raman spectra of initial MWCNTs, ball-milled composite powder, and hot-rolled composite sheet
The grain size of three samples after hot-rolling was analyzed using XRD patterns, as shown in Fig. 3. The nanocrystalline Al specimen clearly shows the peaks of single α(Al) phase. Meanwhile, MWCNTs and Al8CeCu4 peaks, are additionally detected on the XRD patterns of Al-MWCNTs composite and alloy specimens. For the quantitative analysis of the grain growth during hot rolling, the grain sizes of the three specimens before and after hot rolling were calculated using the Scherrer formula with their XRD patterns. As shown in Table 1, the grain sizes of specimens after being hot-rolled are approximately twice those before hot-rolling in nanocrystalline Al and alloy. However, the grain sizes of Al-MWCNTs composite before and after hot-rolling are almost equal to each other, meaning the strong restriction of grain growth during hot-rolling by the MWCNTs.
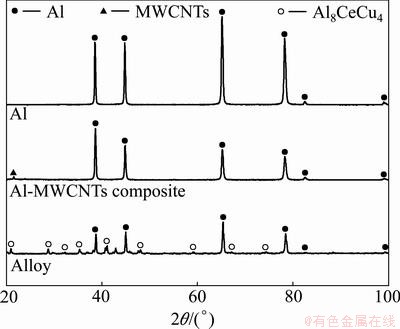
Fig. 3 XRD patterns of Al, Al-MWCNTs composite and alloy after hot-rolling
Table 1 Grain sizes of Al, Al-MWCNTs composite and alloy before and after hot-rolling measured by Scherrer formula from XRD results
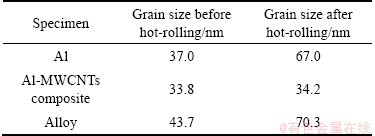
Figure 4 exhibits TEM and HRTEM images of the specimens of nanocrystalline aluminum (Figs. 4(a) and (b)), Al-MWCNTs composite (Figs. 4(c) and (d)), and aluminum alloy (Figs. 4(e) and (f)) after hot-rolling. Most of grains in the nanocrystalline aluminum and alloy show grain sizes ranging from 50 to 150 nm, as shown in Figs. 4(a) and (e). On the other hand, the composite shows much smaller grains with sizes ranging from ~20 to 100 nm. This observation is well matched with grain size measurement based on XRD analysis. Aluminum alloy also exhibits a number of spherical type precipitates with sizes ranging from a few to hundreds of nanometers, as shown in Fig. 4(e), which is comparable with the XRD analysis that indicates the formation of a large amount of Al8CeCu4 particles during hot-rolling. In HRTEM image of nanocrystalline aluminum (Fig. 4(b)), two different types of grains are observed: large grains around 50 nm (~95% in volume fraction) and spherical small grains around 10 nm (~5% in volume fraction). The small grains located near the large grains can be developed during the hot rolling process via dynamic recrystallization. However, grains in the monolithic aluminum seem to grow rapidly at such a high temperature overall. In HRTEM image of the composite (Fig. 4(d)), walls of a MWCNT are clearly observed and any void is not detected at the interface between the MWCNT and the aluminum matrix. On the other hand, HRTEM image of the alloy clearly exhibits the precipitates with a size of tens of nanometers where lattice structure is perfectly different from that of the aluminum matrix.
Figure 5 shows the evolution of Vickers hardness of specimens with heat-treatment time. Before heat-treatment, the hardness of the aluminum alloy is found to be the highest among as-rolled specimens. Hence, precipitation is thought to be more effective for strengthening nanocrystalline aluminum as compared with MWCNT dispersion. Indeed, the reinforcing effect of MWCNTs has been reported to be halved as the grain size of the matrix is reduced down to ~70 nm, due to the limited strain field forming around MWCNTs upon loading [31]. However, the hardness of nanocrystalline aluminum and alloy decreases rapidly with heat- treatment time, possibly because of rapid grain growth. On the other hand, the hardness of the composite is rarely reduced (just HV ~20 reduction even after 5 d heat treatment), indicating that MWCNTs are most effective to suppress the grain growth of nanocrystalline aluminum at high temperatures.
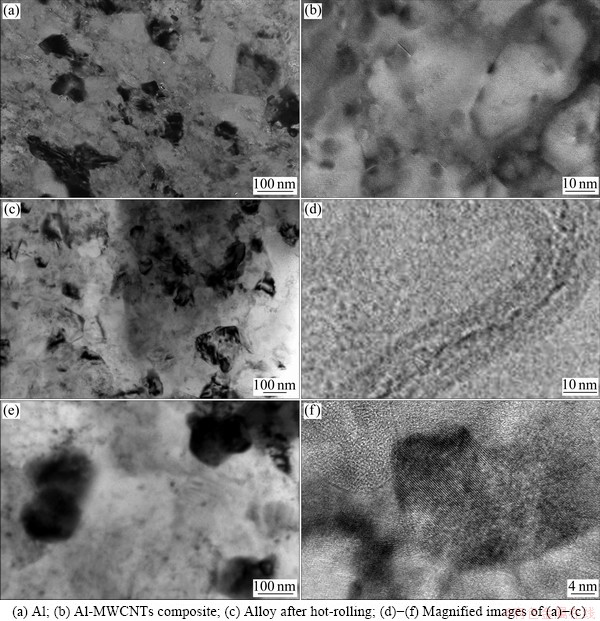
Fig. 4 TEM images
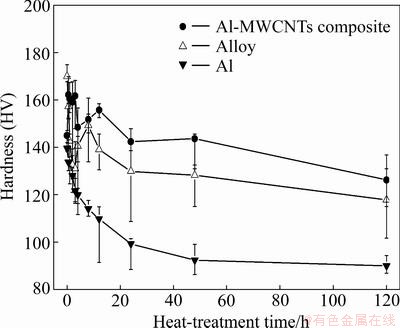
Fig. 5 Evolution of Vickers hardness of three specimens during heat treatment at 550 °C for up to 120 h
4 Conclusions
The grain growth behaviors of nanocrystalline aluminum, alloy and composite containing MWCNTs are compared, by annealing these samples at 550 °C for up to 5 d. The hardness of as-rolled specimens is the highest for nanocrystalline aluminum alloy, meaning that the precipitates are most effective for strengthening nanocrystalline aluminum. The hardness of nanocrystalline aluminum and alloy decreases rapidly as annealing is processed whereas that of nanocrystalline composite almost remains even after 5 d annealing, indicating that MWCNTs are most effective to suppress the grain growth of nanocrystalline aluminum at high temperatures.
Acknowledgement
This work was supported in part by the New Faculty Research Program 2012 of Kookmin University in Korea. Hyunjoo CHOI also acknowledges the support from the Priority Research Centers Program (2012-0006680) and the Korea-Belarus Joint Research Program (2012- 057348) through the National Research Foundation of Korea (NRF) funded by the Ministry of Education, Science and Technology.
References
[1] SCHIMEK M, SPRINGER A, KAIERLE S, KRACHT D, WESLING V. Laser-welded dissimilar steel-aluminum seams for automotive lightweight construction [J]. Physics Procedia, 2012, 39: 43-50.
[2] IMMARIGEON J P, HOLT R T, KOUL A K, ZHAO L, WALLACE W, BEDDOES J C. Lightweight materials for aircraft applications [J]. Materials Characterization, 1995, 35(1): 41-67.
[3] JIA Q J, LIU J Y, LI Y X, WANG W S. Microstructure and properties of electronic packaging box with high silicon aluminum-base alloy by semi-solid thixoforming [J]. Transactions of Nonferrous Metals Society of China, 2013, 23(1): 80-85.
[4] YAMAKOV V, WOLF D, PHILLPOT S R, MUKHERJEE A K, GLEITER H. Dislocation processes in the deformation of nanocrystalline aluminium by molecular-dynamics simulation [J]. Nat Mater, 2002, 1(1): 45-49.
[5] MURAYAMA M, HOWE J M, HIDAKA H, TAKAKI S. Atomic-level observation of disclination dipoles in mechanically milled [J]. Nanocrystalline Fe Science, 2002, 295(5564): 2433-2435.
[6] NIENDORF T, MAIER H J, CANADINC D, YAPICI G G, KARAMAN I. Improvement of the fatigue performance of an ultrafine-grained Nb-Zr alloy by nano-sized precipitates formed by internal oxidation [J]. Scripta Materialia, 2008, 58(7): 571-574.
[7] CHOI H J, KWON G B, LEE G Y, BAE D H. Reinforcement with carbon nanotubes in aluminum matrix composites [J]. Scripta Materialia, 2008, 59(3): 360-363.
[8] CHA S I, KIM K T, ARSHAD S N, MO C B, LEE K H, HONG S H. Field-emission behavior of a carbon-nanotube-implanted Co nanocomposite fabricated from pearl-necklace-structured carbon nanotube/Co powders [J]. Advanced Materials, 2006, 18(5): 553-558.
[9] ZHONG R, CONG H, HOU P. Fabrication of nano-Al based composites reinforced by single-walled carbon nanotubes [J]. Carbon, 2003, 41(4): 848-851.
[10] SANDERS P G, EASTMAN J A, WEERTMAN J R. Elastic and tensile behavior of nanocrystalline copper and palladium [J]. Acta Materialia, 1997, 45(10): 4019-4025.
[11] CHOKSHI A H, ROSEN A, KARCH J, GLEITER H. On the validity of the hall-petch relationship in nanocrystalline materials [J]. Scripta Metallurgica, 1989, 23(10): 1679-1683.
[12] LU K, WEI W D, WANG J T. Microhardness and fracture properties of nanocrystalline Ni-P alloy [J]. Scripta Metallurgica et Materialia, 1990, 24(12): 2319-2323.
[13]
H J, AVERBACK R S. Grain growth in nanocrystalline TiO2 and its relation to vickers hardness and fracture toughness [J]. Scripta Metallurgica et Materialia, 1990, 24(12): 2401-2406.
[14] NOSKOVA N I. The structure and properties of nanocrystalline polyphase alloys [J]. Nanostructured Materials, 1997, 9(1-8): 505-508.
[15] JIA D, WANG Y M, RAMESH K T, MA E, ZHU Y T, VALIEV R Z. Deformation behavior and plastic instabilities of ultrafine-grained titanium [J]. Applied Physics Letters, 2001, 79(5): 611-613.
[16] MCFADDEN S X, MISHRA R S, VALIEV R Z, ZHILYAEV A P, MUKHERJEE A K. Low-temperature superplasticity in nanostructured nickel and metal alloys [J]. Nature, 1999, 398(6729): 684-686.
[17] TANIMOTO H, SAKAI S, MIZUBAYASHI H. Mechanical property of high density nanocrystalline gold prepared by gas deposition method [J]. Nanostructured Materials, 1999, 12(5-8): 751-756.
[18] CHOI H J, LEE S W, PARK J S, BAE D H. Tensile behavior of bulk nanocrystalline aluminum synthesized by hot extrusion of ball-milled powders [J]. Scripta Materialia, 2008, 59(10): 1123-1126.
[19] CHOI H J, LEE S W, PARK J S, BAE D H. Positive deviation from a Hall-Petch relation in nanocrystalline aluminum [J]. Materials Transactions, 2009, 50(3): 640-643.
[20]
K J. Microstructures in the 6060 aluminium alloy after various severe plastic deformation treatments [J]. Materials Characterization, 2011, 62(3): 327-332.
[21] RITTNER M N, WEERTMAN J R, EASTMAN J A, YODER K B, STONED D S. Mechanical behavior of nanocrystalline aluminum- zirconium [J]. Materials Science and Engineering A, 1997, 237(2): 185-190.
[22] VIERKE J, SCHUMACHER G, PILYUGIN V P, DENKS I A, ZIZAK I, WOLF C, WANDERKA N, WOLLGARTEN M, BANHART J. Deformation-induced crystallization in amorphous Al85Ni10La5 alloy [J]. Journal of Alloys and Compounds, 2010, 493(1-2): 683-691.
[23]
J. Alloy effects in nanostructures [J]. Nanostructured Materials, 1993, 3(1-6): 261-272.
[24] KIRCHHEIM R. Grain coarsening inhibited by solute segregation [J]. Acta Materialia, 2002, 50(2): 413-419.
[25] KIRCHHEIM R. Reducing grain boundary, dislocation line and vacancy formation energies by solute segregation: I. Theoretical background [J]. Acta Materialia, 2007, 55(15): 5129-5138.
[26] MILLETT P C, SELVAM R P, SAXENA A. Stabilizing nanocrystalline materials with dopants [J]. Acta Materialia, 2007, 55(7): 2329-2336.
[27] LUO J, CHENG H, ASL K M, KIELY C J, HARMER M P. The role of a bilayer interfacial phase on liquid metal embrittlement [J]. Science, 2011, 333(6050): 1730-1733.
[28] KLUG H P, ALEXANDER L E. X-ray diffraction procedures for polycrystalline and amorphous materials [M]. London: John Wiley and Sons, 1954: 851.
[29] HAYAZAWA N, YANO T, WATANABE H, INOUYE Y, KAWATA S. Detection of an individual single-wall carbon nanotube by tip-enhanced near-field Raman spectroscopy [J]. Chemical Physics Letters, 2003, 376(1-2): 174-180.
[30] TARANTILI P A, ANDREOPOULOS A G, GALIOTIS C. Real-time micro-Raman measurements on stressed polyethylene fibers: 1. Strain rate effects and molecular stress redistribution [J]. Macromolecules, 1998, 31(20): 6964-6976.
[31] CHOI H J, SHIN J H, BAE D H. Grain size effect on the strengthening behavior of aluminum-based composites containing multi-walled carbon nanotubes [J]. Composites Science and Technology, 2011, 71(15): 1699-1705.
纳米铝晶粒生长的抑制方法
Gowoon JEONG, Jooyoung PARK, Singon KANG, Hyunjoo CHOI
School of Advanced Materials Engineering, Kookmin University, 77 Jeongneung-ro, Seongbuk-gu, Seoul, 136-702, Korea
摘 要:对纳米铝、铝合金和铝基化合物的晶粒生长行为进行了比较。首先,采用球磨粉末的烧结方法制备纳米铝。然后,添加能在晶界形成热稳定二次相和阻碍晶界运动的元素,如Mn、Zr和混合稀土元素,形成纳米铝合金。最后,因为多壁碳纳米管有望在晶界处抑制晶粒生长,因此,制备含有多壁碳纳米管的纳米铝复合材料。将3种样品在550 °C,5 d下进行退火处理,比较了退火时间对样品维氏硬度的影响。结果表明,采用多壁碳纳米管阻碍纳米铝晶粒生长的方法最为有效。
关键词:铝(Al);碳纳米管(CNTs);复合材料;纳米晶;晶粒生长
(Edited by Bing YANG)
Corresponding author: Hyunjoo CHOI; Tel: +82-2-910-4287; Fax: +82-2-910-5647; E-mail: hyunjoo@kookmin.ac.kr
DOI: 10.1016/S1003-6326(14)63296-9