
Effects of H2O2 pretreatment on surface characteristics and bioactivity of NaOH-treated NiTi shape memory alloy
CHU Cheng-lin(储成林)1, HU Tao(胡 涛)1, ZHOU Jun(周 俊)1, PU Yue-pu(浦跃朴)2,
YIN Li-Hong(尹立红)2, DONG Yin-sheng(董寅生)1, LIN Ping-hua(林萍华)1,
CHUNG Jonathan-CY(钟志源)3, CHU Paul-K(朱剑豪)3
1. School of Materials Science and Engineering, Southeast University, Nanjing 210096, China;
2. School of Public Health, Southeast University, Nanjing 210096, China;
3. Department of Physics and Materials Science, City University of Hong Kong, Hong Kong, China
Received 15 March 2006; accepted 22 June 2006
Abstract: The effects of H2O2 pretreatment on the surface characteristics and bioactivity of NaOH-treated NiTi shape memory alloy(SMA) were investigated by scanning electron microscopy, X-ray diffraction, X-ray photoelectron spectroscopy, Raman spectra, Fourier transform infrared spectroscopy as well as a simulated body fluid(SBF) soaking test. It is found that the H2O2 pretreatment can lead to the direct creation of more Ti—OH groups and the decrease in the amount of Ni2O3, Na2TiO3 and remnant NiTi phases on the surfaces of bioactive NiTi SMA prepared by NaOH treatment. As a result, the induction period of apatite formation is shortened by dispensing with the slow kinetic formation process of Ti—OH groups via an exchange of Na+ ions from Na2TiO3 phase with H3O+ ions in SBF, which indicates that the bioactivity of NaOH-treated NiTi SMA can be further improved by the H2O2 pretreatment.
Key words: shape memory alloy(SMA); NiTi; NaOH treatment; surface structure; bioactivity; H2O2 pretreatment
1 Introduction
It has been reported that chemical treatment in alkali solutions is an effective method to prepare bioactive titanium metals for medical applications[1-5]. The structural changes of titanium surfaces during alkaline treatment lead to the formation of a bioactive titanate layer containing alkali ions, which can accelerate the spontaneous nucleation of a bonelike apatite layer on Ti surface in simulated body fluid(SBF). Alkaline treatment is also suggested to be effective for inducing the bioactivity of tantalum metal[6]. However, it is not effective for all metallic biomaterials, e.g. SUS316L stainless steel and Co-Cr-Mo alloy[2].
NiTi shape memory alloy(SMA) with unique shape memory effect and superelastic properties has been frequently used as biomedical applications[7, 8]. Note the fact that NiTi SMA contains a large amount of Ni, which can lead to the allergic and toxic responses[9]. Therefore, the surface structure is of interest for its effects on the biocompatibility of NiTi implants[10-14]. As its medical applications become widespread, a more biocompatible, even bioactive NiTi SMA obtained by modifying its surface structure is needed. CHEN et al [15,16] reported that bioactive NiTi SMA could also be obtained by NaOH treatment. The surface structural changes of NiTi SMA during alkali treatment can accelerate the subsequent deposition of apatite layer on NiTi surface in SBF. However, few systematic studies have been reported to date to investigate whether and how the H2O2 pretreatment affects the surface characteristics and the bioactivity of alkali-treated NiTi SMA. This kind of study is very important from a fundamental viewpoint because the bioactivity of alkali- treated NiTi SMA is directly associated with its surface characteristics.
In this work, the native surface oxides on NiTi substrate were removed by chemical polishing pretreatment firstly, and then NiTi substrate was pretreated by H2O2 solution. After subsequent NaOH treatment, the effects of H2O2 pretreatment on the surface characteristics and the bioactivity of NaOH-treated NiTi SMA were investigated by scanning electron microscopy (SEM), X-ray diffraction(XRD), X-ray photoelectron spectroscopy(XPS), Raman spectra, Fourier transform infrared spectroscopy(FTIR) as well as a SBF soaking test.
2 Experimental
A commercially available NiTi (50.8% Ni, mole fraction) SMA plate for medical applications was cut into small rectangular blocks (10 mm×10 mm×1 mm). All samples were chemically polished to remove the native surface oxides for 10 min in Kroll’s reagent: a mixture of 2 mL hydrofluoric acid (HF, 40%), 4 mL nitric acid (HNO3, 40%) and 994 mL deionized water. The samples were subsequently ultrasonically washed in acetone for 10 min and in deionized water for 10 min. They were divided into two groups. The first group was used as control (denoted as the chemically-polished NiTi SMA). The second group was subsequently pretreated in a boiling aqueous solution containing 30%H2O2 for 2 h (denoted as the H2O2-pretreated NiTi SMA). After that, both the chemically-polished one and the H2O2- pretreated one were finally treated in 10 mol/L NaOH aqueous solution at 60 ℃ for 24 h.
After ultrasonically washed in acetone and rinsed in deionized water, all NaOH-treated samples were soaked in a SBF for 12 h and 24 h. The SBF solution was buffered at pH 7.4 with trimethanol aminomethane-HCl. The ionic concentrations in the SBF solution are nearly equal to those in human body blood plasma and are (mmol/L): Na+ 142.0, K+ 5.0, Ca2+ 2.5, Mg2+ 1.5,
4.2, Cl- 148.5,
1.0 and
0.5.
The samples were XPS analyzed using a VG Scientific ESCALAB 5 spectrometer with monochroma- tic Al Ka(1 486.6 eV) X-ray radiation. Survey spectra in the range of 400-1 200 eV were recorded for each sample at a 50 eV constant pass energy, followed by higher resolution spectra over Ti 2p, Ni 2p, O 1s and Na 1s ranges using a 20 eV pass energy, which were used for assessment of the chemical state as well as for quantification. XRD patterns were taken with an X-ray diffractometer (RAD IIA, Rigaku, Japan) operated with Cu Kα under 40 kV and 25 mA, equipped with a thin- film attachment on which the glancing angle was 1?. Raman scattering spectra were recorded with a Renishaw system 2000 spectrometer, using the 514 nm line of Ar+ for excitation. The light beam was focused to a spot of about 5 mm in size. FTIR spectra were recorded using FTIR spectrometry (Nicolet 800). The surface morphology was observed by a Philips XL30 FEG SEM after the surfaces were coated with gold films.
3 Results
Fig.1 shows the SEM photographs of the surface of the H2O2-pretreated NiTi SMA in comparison with the chemically-polished NiTi SMA. Many pores are observed on the chemically-polished NiTi SMA, whose surface concentration of oxygen as indicated by XPS element analysis (not shown here) is only about 7.9% (mole fraction), which suggests that the native surface oxides on NiTi SMA have been removed by chemical polishing treatment. After NiTi SMA is pretreated with H2O2 solution, an oxide layer with some microcracks is formed on its surface. The XRD patterns (Fig.2) further indicate that this oxide layer is mainly composed of rutile and anatase TiO2 phases.
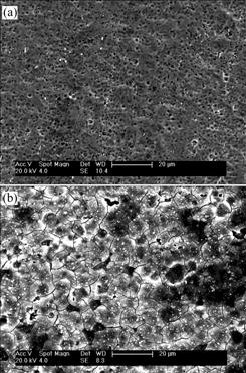
Fig.1 SEM images of surfaces of different NiTi SMAs: (a) Chemically-polished; (b) H2O2-pretreated
Fig.3 shows the SEM images of the surfaces of two NiTi SMAs (the chemically-polished one and the H2O2-pretreated one) after subsequent NaOH treatment. Both of them are covered by porous surface layers with flake structure, however, the flake-shape substance on the former has a sharper edge, while the one on the latter
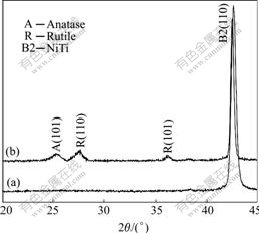
Fig.2 XRD spectra of surfaces of different NiTi SMAs: (a) Chemically-polished; (b) H2O2-pretreated
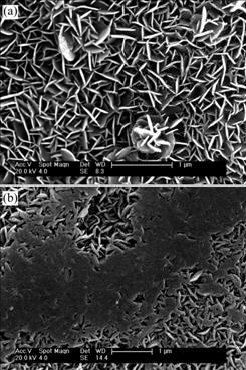
Fig.3 SEM images of surfaces of different NiTi SMAs after subsequent NaOH treatment: (a) Chemically-polished; (b) H2O2-pretreated
has a blunt rim and is relatively denser. Fig.4 shows the typical XPS spectra of two samples. The surface concentrations of Na, Ni and O are 12.4%, 29.4%, 32.6% for the former, and 5.0%, 5.3%, 58.8% for the latter, respectively. The results indicate that the H2O2 pretreatment can decrease remarkably the content of Na and Ni in the surface structure of NaOH-treated NiTi SMAs.
Fig.5 shows the XRD patterns of the surfaces of two NiTi SMAs after NaOH treatment. Besides NiTi sub-
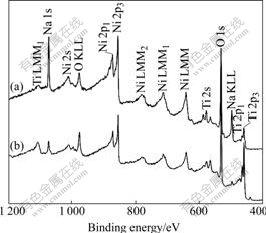
Fig.4 XPS spectra of surfaces of different NiTi SMAs after subsequent NaOH treatment: (a) Chemically-polished; (b) H2O2-pretreated
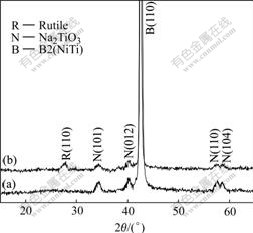
Fig.5 XRD spectra of surfaces of different NiTi SMAs after subsequent NaOH treatment: (a) Chemically-polished; (b) H2O2-pretreated
strate phase, a sodium titanate (Na2TiO3) presents on the surface. This reveals that NaOH-treatment can result in the formation of sodium titanate layer on both the chemically-polished NiTi SMA and the H2O2-pretreated one as it does for pure titanium[1-5]. However, rutile TiO2 is also found on the H2O2-pretreated one after subsequent NaOH treatment. The crystalline of porous surface structures of two samples is relatively low as indicated by the widened XRD peaks with low intensities in Fig.5.
The Raman spectra of the surfaces of two NiTi SMAs after NaOH treatment were recorded and are presented in Fig.6. For both samples, the characteristic air bands are visible at low wave numbers up to (180 ± 10)cm-1[17]. The existence of Ti—O groups correspond- ing to Na2TiO3 on the chemically-polished one after NaOH treatment is confirmed by the presence of bands at 220-280 cm-1. More Ti—O groups corresponding to TiO2 and Na2TiO3 on the H2O2-pretreated one after subsequent NaOH treatment are confirmed by the presence of bands at 220-280 cm-1 and (447±10) cm-1. The broadening of these bands suggests the relatively low crystalline of Na2TiO3 or TiO2, which is consistent with the XRD result (Fig.5). The band near 705 cm-1 in the spectrum (Fig.6(b)) may come from the defects in rutile TiO2 crystal[18].
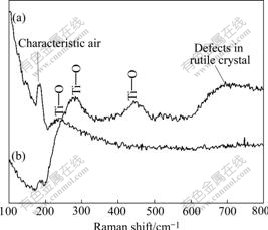
Fig.6 Raman spectra of surfaces of different NiTi SMAs after subsequent NaOH treatment: (a) Chemically-polished; (b) H2O2-pretreated
Figs.7(a) and (b) show the high resolution Ti 2p and Ni 2p XPS spectra of the surfaces of two NiTi SMAs after NaOH treatment, respectively. It can be seen from Ti 2p spectra that there are two dominant peaks for both samples, which is identified as Ti4+ (Ti—O) 2p3/2 at 459.3 eV and Ti4+ (Ti—O) 2p1/2 at 464.8 eV. A small peak near 454.5 eV corresponding to TiNi-Ti 2p3/2 spin states is found on the curve for the chemically polished NiTi SMA after NaOH treatment. Ni 2p spectra of both samples mainly consist of two major peaks at 853.8 eV and 870.5 eV, which are assigned to NiNi-Ti 2p3/2 and 2p1/2 in intermetallic NiTi state, respectively. NiNi-Ti 2p spectra exhibit a satellite structure, which is separated from the main peaks by about 7 eV. In addition, the small Ni3+ (Ni2O3) 2p3/2 and 2p1/2 peaks at 856.2 eV and 873.9 eV are also present, especially in the XPS spectrum for the chemically polished NiTi SMA after NaOH treatment. As indicated by the intensities of TiNi-Ti 2p3/2 (Fig.7(a)) and Ni3+ 2p3/2 (Fig.7(b)) peaks, the amount of remnant NiTi phase and Ni2O3 on the NaOH-treated NiTi SMAs is reduced by the H2O2 pretreatment. O 1s XPS spectra of two NiTi SMAs after NaOH treatment are shown in Fig.7(c). The peaks assigned to oxygen present in metal
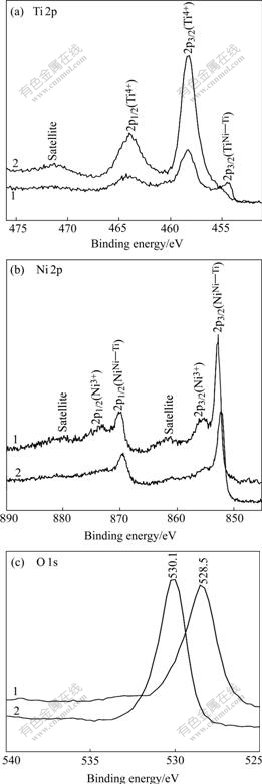
Fig.7 XPS spectra of surfaces of different NiTi SMAs after subsequent NaOH treatment: 1 Chemically-polished; 2 H2O2- pretreated oxides for both samples are different, whose binding energies are near 528.5 eV for the chemically-polished NiTi SMA after NaOH treatment and 530.1 eV for the H2O2-pretreated one after subsequent NaOH treatment. The reason for this difference of O 1s peak is unclear and needs future studies.
The results of FTIR spectroscopic measurements are shown in Fig.8. In comparison with the spectrum of the surface of chemically-polished NiTi SMA after NaOH treatment, the one for the H2O2-pretreated one after subsequent NaOH treatment has a broader and stronger OH- stretching bands from 3 700 cm-1 to 2 500 cm-1 derived from Ti—OH groups[19,20], which indicates that the H2O2 pretreatment can result in the direct creation of more Ti—OH groups in the porous surface structure of the NaOH-treated NiTi SMAs.
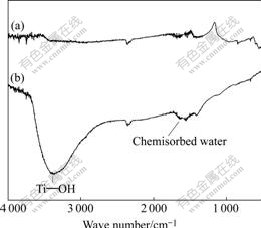
Fig.8 FTIR spectra of surfaces of different NiTi SMAs after subsequent NaOH treatment: (a) Chemically-polished; (b) H2O2-pretreated
Fig.9 depicts SEM images of the apatites deposited on the H2O2-pretreated one after subsequent NaOH treatment in SBF for 12 h and 24 h. After 12 h immersion in SBF, some single and clustered ball-like apatites are observed (Fig.9(a)). After an immersion time of 24 h, the number and size of these ball-like apatite particles increase. In contrast, no apatites can be found on the chemically polished NiTi SMA after NaOH treatment in SBF for 24 h (not shown here), which indicates that the induction period of apatite formation on the NaOH-treated NiTi SMAs can be shortened by the H2O2 pretreatment.
4 Discussion
The results from XRD, Raman spectra, FTIR and XPS reveal that the H2O2 pretreatment can lead to the direct creation of more Ti—OH groups and the decrease in the amount of Ni2O3, Na2TiO3 and remnant NiTi phase in the porous surface structure of NaOH-treated NiTi SMAs. As a consequence, the induction period of apatite formation on the NaOH-treated NiTi SMAs is shortened as confirmed by the SBF soaking test, which indicates that the bioactivity of NaOH-treated NiTi SMAs can be further improved by the H2O2 pretreatment.
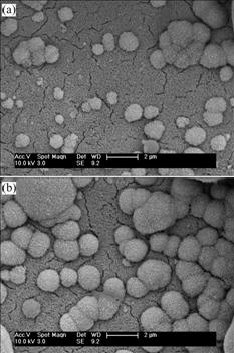
Fig.9 SEM images of apatites deposited on H2O2-pretreated NiTi SMA after subsequent NaOH treatment in SBF for different times: (a) 12 h; (b) 24 h
It is well known that sodium titanate phases on NaOH-treated titanium can result in the formation of Ti—OH groups via an exchange of Na+ ions with H3O+ ions in SBF[1-5]. Ti—OH groups are generally accepted as one of the key factors for apatite formation and the nucleation rate of apatites on NaOH-treated titanium is mainly controlled by the formation process of Ti—OH groups[21]. However, the formation of Ti—OH groups via the exchange of Na+ ions from sodium titanate with H3O+ ions in SBF is a slow kinetic process, especially when sodium titanate phase is well crystallized. In this work, although the H2O2 pretreatment can reduce the amount of sodium titanate (Na2TiO3), the direct creation of more Ti—OH groups on the surface of the H2O2- pretreated NiTi after subsequent NaOH treatment is also beneficial for its bioactivity and can decrease the induction period of apatite formation by dispensing with the slow kinetic formation process of Ti—OH groups.
5 Conclusions
The H2O2 pretreatment can lead to the direct creation of more Ti—OH groups and the decrease in the amount of Ni2O3, Na2TiO3 and remnant NiTi phase in the porous surface structure of NaOH-treated NiTi SMA. As a consequence, the induction period of apatite formation on the bioactive NiTi SMA prepared by NaOH treatment is shortened by dispensing with the slow kinetic formation process of Ti—OH groups via an exchange of Na+ ions from sodium titanate with H3O+ ions in SBF. Thus the bioactivity of NaOH-treated NiTi SMA can be further improved by the H2O2 pretreatment.
References
[1] Kokubo T, Miyaji F, Kim H M, Nakamura T. Spontaneous formation of bonelike apatite layer on chemically treated titanium metals [J]. J Am Ceram Soc, 1996, 79: 1127-1129.
[2] Kim H M, Miyaji F, Kokubo T, Kitsugi T, Nakamura T. Preparation of bioactive Ti and its alloys via simple chemical surface treatment [J]. J Biomed Mater Res, 1996, 32: 409-417.
[3] Yan W Q, Nakamura T, Kawanabe K, Nishiguchi S, Oka M, Kokubo T. Apatite layer-coated titanium for use as bone bonding implants [J]. Biomaterials, 1997, 18: 1185-1190.
[4] Kim H M, Miyaji F, Kokubo T, Nishiguchi S, Nakamura T. Graded surface structure of bioactive titanium prepared by chemical treatment [J]. J Biomed Mater Res, 1999, 45: 100-107.
[5] Jonasova L, Muller F A, Helebrant A, Strnad J, Greil P. Biomimetic apatite formation on chemically treated titanium [J]. Biomaterials, 2004, 25: 1187-1194.
[6] Miyazaki T, Kim H M, Miyaji F, Kokubo T, Kato H, Nakamura T. Bioactive tantalum metal prepared by NaOH treatment [J]. J Biomed Mater Res, 2000, 50: 35-42.
[7] Duerig T, Pelton A, Stockel D. An overview of nitinol medical applications [J]. Mater Sci Eng A, 1999, 149: 273-275.
[8] Otsuka K, Wayman C M. Shape Memory Materials [M]. Cambridge: Cambridge University Press, 1998.
[9] Ryhanen J, Kallioinen M, Tuukkanen J. Bone modeling and cell-material interface responses induced by nickel-titanium shape memory alloy after periosteal implantation [J]. Biomaterials, 1999, 20: 1309-1317.
[10] Ratner B D, Hoffman A S, Schoen F J, Lemons J E. Biomaterials Science [M]. London: Academic Press, 1996.
[11] Wever D J, Veldhuizen A G, Vries J de, Busscher H J, Uges D R A, Horn J R van. Electrochemical and surface characterization of a nickel-titanium alloy [J]. Biomaterials, 1998, 19: 761-769.
[12] Rondelli G, Vicentini B. Localized corrosion behaviour in simulated human body fluids of commercial Ni-Ti orthodontic wires [J]. Biomaterials, 1999, 20: 785-792.
[13] Shabalovskaya S A, Anderegg J W. Surface spectroscopic characterization of TiNi nearly equiatomic shape memory alloys for implants [J]. J Vac Sci Technol A, 1995, 13(5): 2624-2632.
[14] Filip P, Lausmaa J, Musialek J, Mazanec K. Structure and surface of TiNi human implants [J]. Biomaterials, 2001, 22: 2131-2138.
[15] Chen M F, Yang X J, Liu Y, Zhu S L, Cui Z D, Man H C. Study on the formation of an apatite layer on NiTi shape memory alloy using a chemical treatment method [J]. Surface and Coatings Technology, 2003, 173: 229-234.
[16] Chen M F, Yang X J, Hu R X, Cui Z D, Man H C. Bioactive NiTi shape memory alloy used as bone bonding implants [J]. Mater Sci Eng C, 2004, 24: 497-502.
[17] Pino A P, Serra P, Morenza J L. Coloring of titanium by pulsed laser processing in air [J]. Thin Solid Films, 2002, 415: 201-205.
[18] Li X, Kobayashi T, Sekine T. Stability of TiN and fast synthesis of rutile from TiN and CuO by shock compression [J]. Solid State Communications, 2004, 130: 79-82.
[19] Music S, Gotic M, Ivanda M, Popovic S, Turkovic A, Trojko R, Sekulic A, Furic K. Chemical and microstructural properties of TiO2 synthesized by sol-gel procedure [J]. Mater Sci Eng B, 1997, 47: 33-40.
[20] Skryshevskyy V A, Dittrich Th, Rappich J. Infrared-active defects in a TiO2 mixture of coexisting anatase and rutile phases [J]. Phys Stat Sol A, 2004, 201(1): 157-161.
[21] Li P, Ohtsuki C, Kokubo T, Nakanishi K, Soga N, DeGroot K. The role of hydrated silica, titania and alumina in inducing apatite on implants [J]. J Biomed Mater Res, 1994, 28: 7-15.
(Edited by YUAN Sai-qian)
Foundation item: Project(50501007) supported by the National Natural Science Foundation of China; Project(BK2003062) supported by the Natural Science Foundation of Jiangsu Province, China; Project(CityU 1/04C) supported by Hong Kong Research Grants Countil(RGC) Central Allocation Group Research; Project(4012001007) supported by Teaching and Research Award Program for Outstanding Young Teachers of Southeast University; Project(9212001352) supported by Pre-research Project for National Natural Science Foundation in Southeast University, China
Corresponding author: CHU Cheng-lin; Tel: +86-25-83996387; E-mail: clchu@seu.edu.cn