Trans. Nonferrous Met. Soc. China 23(2013) 731-734
Rapid solidification of Cu60Co30Cr10 alloy under different conditions
Jin-bo GUO1, Chong-de CAO1, Su-lian GONG1, Rui-bo SONG1, Xiao-jun BAI1, Jian-yuan WANG1, Jian-bang ZHENG1, Xi-xing WEN1, Zhan-bo SUN2
1. Key Laboratory of Space Applied Physics and Chemistry of Ministry of Education, Department of Applied Physics, Northwestern Polytechnical University, Xi’an 710072, China;
2. Key Laboratory for Non-equilibrium Synthesis and Modulation of Condensed Matter, Ministry of Education, Xi’an Jiaotong University, Xi’an 710049, China
Received 29 December 2011; accepted 17 February 2012
Abstract: Metastable liquid phase separation and rapid solidification in a metastable miscibility gap were investigated on the Cu60Co30Cr10 alloy by using the electromagnetic levitation and splat-quenching. It is found that the alloy generally has a microstructure consisting of a (Co,Cr)-rich phase embedded in a Cu-rich matrix, and the morphology and size of the (Co,Cr)-rich phase vary drastically with cooling rate. During the electromagnetic levitation solidification processing the cooling rate is lower, resulting in an obvious coalescence tendency of the (Co,Cr)-rich spheroids. The (Co,Cr)-rich phase shows dendrites and coarse spheroids at lower cooling rates. In the splat quenched samples the (Co,Cr)-rich phase spheres were refined significantly and no dendrites were observed. This is probably due to the higher cooling rate, undercooling and interface tension.
Key words: Cu-Co-Cr alloy; rapid solidification; metastable liquid phase separation; electromagnetic levitation; splat-quenching
1 Introduction
Rapid solidification processing might affect several alloy properties, such as microstructural refinement, extension of solid solubility or formation of non-equilibrium phases. This, in turn, may improve the strength, increase plasticity, extend fatigue life, enhance stress corrosion resistance and affect some other related properties of alloys. NAKAGAWA [1] revealed for the first time the miscibility gap in Co-Cu and Fe-Cu systems when measuring their magnetic susceptibility during successive cooling. Since then many efforts have been made to investigate the liquid phase separation and rapid solidification in both binary and relevant ternary systems by different experimental methods, such as Cu-Co [2], Cu-Cr [3], Cu-Ta [4], Cu-Co-Fe [5], Cu-Co-Ni [6,7] systems. For binary alloy, the thermodynamic properties of the liquid phase of the Co-Cu system were determined by TIMBERG et al [8], TASKINEN [9], and TURCHANIN [10]. ELDER et al [11] experimentally determined the position of the Cu-Co miscibility gap by processing a range of samples with different nominal compositions using electro- magnetic levitation and studied the composition by electron microprobe analysis. They also carried out a preliminary work to model the phase diagram considering both stable and metastable equilibria. CAO et al [12-14] successfully undercooled Cu-Co alloys over a composition range of 16.0%-87.2% Cu (mole fraction) and directly measured the liquidus and critical liquid-phase separation temperatures using differential thermal analysis in combination with glass fluxing method. The currently accepted Cu-Cr phase diagram was proposed by JACOB et al [15] following a thermodynamic study of the system using the Knudsen cell-mass spectrometry. It falls into the category of simple eutectic, but includes a metastable miscibility gap in the undercooled liquid. Under the assumption of a pseudo-subregular solution model, they calculated the metastable phase boundaries of the system, and predicted that the miscibility gap has a critical composition of 43.6% Cr (mole fraction) at 1787 K and expands considerably at lower temperatures.
The information about ternary systems is scarce. MUNITZ [16] and CURIOTTO et al [5] studied the liquid phase separation behaviors of Cu-Co-Fe ternary system and found that the alloy melt would separate into (Co,Fe)-rich L1-phase and Cu-rich L2-phase when it was undercooled into the metastable miscibility gap. CAO and
[17] precisely measured the metastable miscibility gap of the Cu-Co-Fe ternary system. SUN et al [18] observed that additional Ni can improve giant magneto resistive (GMR) behavior of the melt-spun Cu-Co ribbons. The reason was that Ni can suppress the liquid phase separation at high temperatures and increase the density of magnetic Co-precipitates. In the case of Cu-Co-Cr system, the interaction among the components is different from that of Cu-Co-Fe or Cu-Co-Ni system. So far, the liquid phase separation and solidification behaviors of Cu-Co-Cr ternary alloys remain unclear. In this work, we aim at exploring the metastable liquid phase separation of Cu60Co30Cr10 alloy under different conditions by the electromagnetic levitation (EML) and splat-quenching techniques.
2 Experimental
Samples with nominal composition of Cu60Co30Cr10 were prepared by arc-melting under Ar atmosphere from high purity copper (99.999%), cobalt (99.95%) and chromium (99.95%). The mass of one sample was about 1 g. After being evacuated to a vacuum pressure of 1×10-4 Pa, the levitation chamber was backfilled with pure argon (99.999% purity). The temperature of the molten sample was measured with a pyrometer. After the sample was melted and overheated, the sample was cooled by blowing pure helium gas (99.999% purity). The sample solidified spontaneously or was splat quenched onto a polished copper plate at a certain temperature. The microstructures of the solidified samples were examined by an optical microscope (Zeiss Axio Imager A1m) and a scanning electron microscope (Zeiss SUPPA55). An X’pert PRO MPD X-ray diffractometer was used to analyze the phase constitution in the solidified ternary alloy.
3 Results and discussion
Figure 1 presents a temperature—time curve of a Cu60Co30Cr10 alloy sample processed by EML. The solid sample was heated and the (Cu) solid solution was melted near 1557 K. Subsequently, the (Co,Cr)-rich phase was gradually melted. The sample completely transformed into liquid at the liquidus temperature (TL) of 1721 K. The alloy was then cooled after homogenization at a temperature above the melting point. There was an evident exothermic signal at 1646 K, which demonstrates the metastable phase separation. Then the separated liquids were solidified under 1646 K.
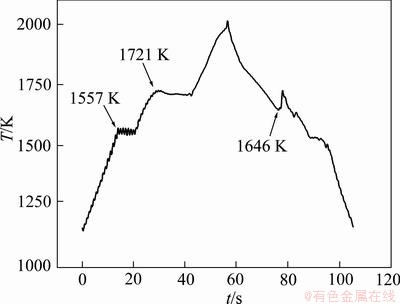
Fig. 1 Temperature (T)—time (t) curve of Cu60Co30Cr10 alloy processed by EML
X-ray diffraction was employed to identify the phase constitution in each sample. Figure 2 shows the XRD patterns of the Cu60Co30Cr10 alloy processed by EML and splat-quenching. The samples processed via both methods exhibit similar phase constitution. The main phases are (Cu) and (CoCr) solid solutions. Some (Co) phase was also formed, but no (Cr) phase was detected. It can be inferred from the XRD patterns that more (Co) solid solution phases were formed in the splat-quenched sample.
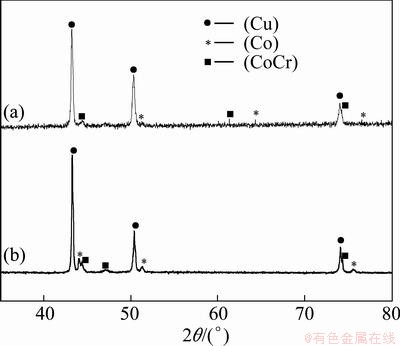
Fig. 2 XRD patterns of Cu60Co30Cr10 alloy processed by EML (a) and splat-quenching (b)
The microstructures suggest the phase transformation path during cooling. Figure 3 shows the optical microscopy image and SEM images of the Cu60Co30Cr10 alloy processed by EML. The dark phase corresponds to (Co,Cr)-rich phase, and the bright matrix is Cu-rich phase. On the sample surface some Cu-rich phase forms a shell. The (Co,Cr)-rich spheroids show an obvious coalescence tendency, where many small liquid spheres form big ones through collision and coagulation. It can be observed that the (Co,Cr)-rich droplets are characterized by irregular shapes. This probably results from the electromagnetic stirring in EML. Within the (Co,Cr)-rich spheres, small Cu-rich spheres can be observed. Inside the Cu-rich liquid the (Co,Cr)-rich was solidified as dendrites. The (Co,Cr)-rich dendrites nucleated preferentially on the surface of the (Co,Cr)- rich spheres and grew outwards. Such a feature in the microstructure implies that the (Co,Cr)-rich dendrites are formed later than the (Co,Cr)-rich spheres, which further provides an evidence for liquid phase separation.
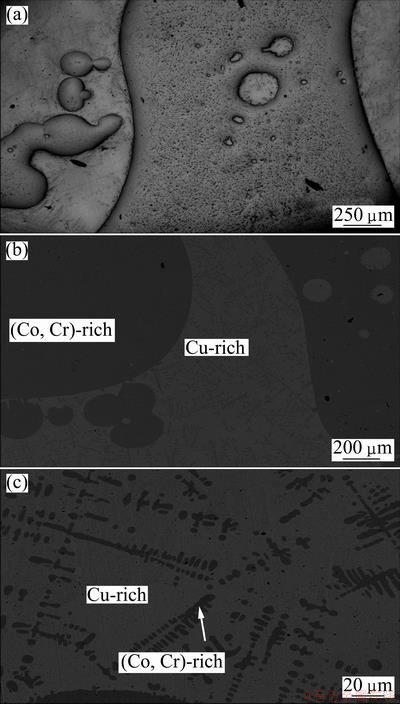
Fig. 3 Optical microscopy image (a) and SEM images (b, c) of Cu60Co30Cr10 alloy processed by EML
Figure 4 shows the SEM images of Cu60Co30Cr10 alloy processed by splat-quenching. In general, two-layer structures were formed with Cu-rich (bright) phase segregating on the free surface and (Co,Cr)-rich (dark) on the chill surface. Possible reasons are the smaller surface tension and lower viscosity of Cu-rich liquid. No dendrite of (Co,Cr)-rich phase is formed from the view of the whole cross-section of the sample. This is probably caused by a high undercooling and large interface energy between the two liquid phases. At a higher undercooling the Cu-rich liquid contains less Co and Cr, which is confirmed by energy dispersive X-ray spectroscopy analysis. A large interface energy probably suppresses the nucleation and growth of (Co,Cr)-rich phase. The cooling rate during splat-quenching is much larger than that in electromagnetic levitation solidification. Consequently, there is less time for the small (Co,Cr)-rich droplets to coalescence before solidification and disperse structures are formed (Figs. 4(a) and (b)). There exists secondary precipitation of Cu-rich phase in the (Co,Cr)-rich spheres and the size of the Cu-rich phase is quite small.
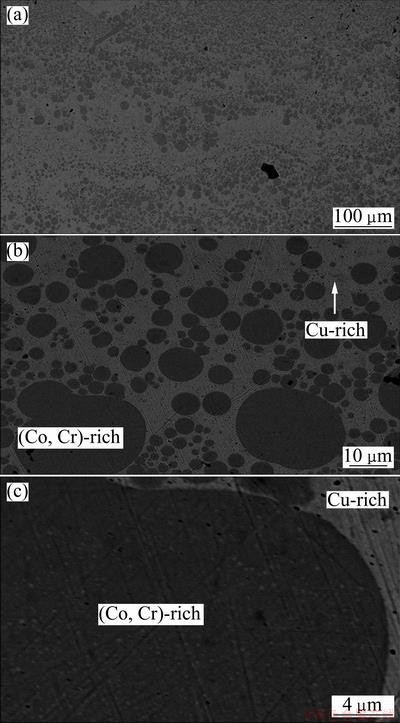
Fig. 4 SEM images of Cu60Co30Cr10 alloy processed by splat-quenching
4 Conclusions
1) Liquid phase separation in a metastable miscibility gap was investigated on the Cu60Co30Cr10 alloy by the EML and splat-quenching techniques. The XRD results reveal that (Cu), (Co) and (CoCr) phases were formed during the EML and splat-quenching solidification processes. The microstructures indicate that metastable liquid phase separation took place in the EML and splat-quenching cases. The microstructures of the samples solidified by splat-quenching are significantly finer than those of the samples solidified during levitation.
2) It is inferred that different cooling rate and undercooling during the two processes play the main dominant in solidification microstructure formation.
References
[1] NAKAGAWA Y. Liquid immiscibility in copper-cobalt systems in the supercooled state. [J]. Acta Metall, 1958, 6: 704-711.
[2] KOLBE M, GAO J R. Liquid phase separation of Co-Cu alloys in the metastable miscibility gap [J]. Materials Science and Engineering A, 2005, 413-414: 509-513.
[3] ZHOU Z M, GAO J R, LI F, ZHANG Y K, WANG Y P, KOLBE M. On the metastable miscibility gap in liquid Cu-Cr alloys [J]. J Mater Sci, 2009, 44: 3793-3799.
[4] GONG H R, KONG L T, LAI W S, LIU B X. Metastable phase formation in an immiscible Cu-Ta system studied by ion-beam mixing, ab initio calculation, and molecular dynamics simulation [J]. Acta Materialia, 2003, 51: 3885-3893.
[5] CURIOTTO S, BATTEZZATI L, JOHNSON E, PALUMBO M, PRYDS N. The liquid metastable miscibility gap in the Cu-Co-Fe system [J]. J Mater Sci, 2008, 43: 3253-3258.
[6] ZANG D Y, WANG H P, DAI F P, LANGEVIN D, WEI B. Solidification mechanism transition of liquid Co-Cu-Ni ternary alloy [J]. Appl Phys A, 2011, 102: 141-145.
[7] CURIOTTO S, BATTEZZATI L, JOHNSON E, PRYDS N. Thermodynamics and mechanism of demixing in undercooled Cu-Co-Ni alloys [J]. Acta Materialia, 2007, 55: 6642-6650.
[8] TIMBERG L, TOGURI J M, AZAKAMI T. A thermodynamic study of copper-iron and copper-cobalt liquid alloys by mass spectrometry [J]. Metall Trans B, 1981, 12: 275-279.
[9] TASKINEN P. Activities and thermodynamic properties of molten Co-Cu alloys [J]. Z Metallkd, 1982, 73: 445-450.
[10] TURCHANIN M A. Enthalpies of solution of titanium, zirconium, and hafnium in liquid copper [J]. Journal of Alloys and Compounds, 1996, 236: 236-242.
[11] ELDER S P, MUNITZ A, ABBASCHIAN G J. Metastable liquid immiscibility in Fe-Cu and Co-Cu alloys [J]. Mater Sci Forum, 1991, 50: 137-150.
[12] CAO C D, HERLACH D M, KOLBE M, GORLER G P, WEI B. Rapid solidification of Cu84Co16 alloy undercooled into the metastable miscibility gap under different conditions [J]. Scripta Materialia, 2003, 48: 5-9.
[13] CAO C D, WEI B. Disperse structures of undercooled Co-40wt% Cu droplets processed in drop tube [J]. J Mater Sci Lett, 2002, 21: 341-343.
[14] CAO C D,
G P, HERLACH D M, WEI B. Liquid-liquid phase separation in undercooled Co-Cu alloys [J]. Mater Sci Eng A, 2002, 325: 503-510.
[15] JACOB K T, PRIYA S, WASEDA Y. Measurement of the activity of boron in liquid copper using a four-phase equilibrium technique [J]. Metallurgical and Materials Transactions A, 2000, 31: 2675-2678.
[16] MUNITZ A. Liquid separation in Cu-Co and Cu-Co-Fe alloys solidified at high cooling rates [J]. Journal of Materials Science, 1998, 33: 3639-3649.
[17] CAO C D,
G P. Direct measurement of the metastable liquid miscibility gap in Fe-Co-Cu ternary alloy system [J]. Chinese Physics Letters, 2005, 22: 482-484.
[18] SUN Z B, SONG X P, HU Z D, LIANG G Y, YANG S, COCHRANE R F. Effects of rare earth additions on GMR of melt-spun Cu-Co-Ni ribbons [J]. Journal of Magnetism and Magnetic Materials, 2001, 234: 279-283.
不同冷却条件下Cu60Co30Cr10合金的快速凝固
郭晋波1,曹崇德1,弓素莲1,宋瑞波1,白晓军1,王建元1,郑建邦1,文喜星1,孙占波2
1. 西北工业大学 应用物理系 空间应用物理与化学教育部重点实验室,西安 710072;
2. 西安交通大学 物质非平衡合成与调控教育部重点实验室,西安 710049
摘 要:利用电磁悬浮和快淬实验研究Cu60Co30Cr10合金在亚稳不混溶区的液相分离和快速凝固特征。结果表明,合金的显微组织为富(Co,Cr)相分布在富Cu相基体中,且富(Co,Cr)相颗粒的形状和大小随着冷却速率的变化而有明显的区别。在悬浮凝固条件下冷却速率较低,富(Co,Cr)相较粗大,有明显的聚集粗化趋势,富(Cu)相中有大量富(Co,Cr)相枝晶。而在快淬凝固条件下富(Co,Cr)相明显细化,富(Cu)相中未发现富(Co,Cr)相枝晶形成,这可能与较高的冷却速率、较大的过冷度和较高的界面张力有关。
关键词:Cu-Co-Cr合金;快速凝固;亚稳液相分离;电磁悬浮;快淬
(Edited by Xiang-qun LI)
Foundation item: Projects (51171152, 50871088) supported by the National Natural Science Foundation of China; Project (20126102110048) supported by Doctoral Fund of Ministry of Education of China; Project (SKLSP201202) supported by Foundation of State Key Laboratory of Solidification, China; Project (2012JC2-02) supported by Natural Science Basic Research Plan in Shaanxi Province, China; Project (JC201268) supported by the NPU Foundation for Fundamental Research, China
Corresponding author: Chong-de CAO; E-mail: caocd@nwpu.edu.cn
DOI: 10.1016/S1003-6326(13)62522-4