Trans. Nonferrous Met. Soc. China 24(2014) 3917-3921
SnO2-based gas (hydrogen) anodes for aluminum electrolysis
Sai-jun XIAO1,2, Tommy MOKKELBOST3, Ove PAULSEN3, Arne P. RATVIK2, Geir M. HAARBERG2
1. School of Metallurgy Engineering, Anhui University of Technology, Maanshan 243002, China;
2. Department of Materials Science and Engineering, Norwegian University of Science and Technology, Trondheim 7491, Norway;
3. SINTEF Materials and Chemistry, Trondheim 7465, Norway
Received 17 October 2013; accepted 17 November 2014
Abstract: A novel SnO2-based gas anode was developed for aluminum electrolysis in molten cryolite at 850 °C to reduce energy consumption and decrease CO2 emissions. Hydrogen was introduced into the anode, participating in the anode reaction. Carbon and aluminum were used as the cathode and reference electrodes, respectively. Cyclic voltammetry was applied in the cell to investigate the electrochemical behavior of oxygen ion on platinum and SnO2-based materials. The potential for oxygen evolution on these electrode materials was determined. Then, galvanostatic electrolysis was performed on the gas anode, showing a significant depolarization effect (a decrease of ~0.8 V of the anode potential) after the introduction of hydrogen, compared with no gas introduction or the introduction of argon. The results indicate the involvement of hydrogen in the anode reaction (three-phase-boundary reaction including gas, electrolyte and electrode) and give the possibility for the utilization of reducing gas anodes for aluminum electrolysis.
Key words: SnO2-based gas anode; hydrogen anode; aluminum electrolysis
1 Introduction
Aluminum and aluminum alloys are very important materials for a wide range of applications in different areas such as transportation, construction and packaging. Primary aluminum was produced in the Hall-Héroult process, which was invented in 1886 [1]. In this process a consumable carbon anode is used which evolves CO2 and must be adjusted and replaced periodically, resulting in disturbing the cell performance and increasing the corresponding energy consumption [1]. Because of the disadvantages of using a consumable carbon anode, non- consumable (inert) anodes for oxygen evolution have been investigated during the last 40 years. When using inert anodes, the anode is dimensionally stable and the need to adjust and change the anode is eliminated. Thus, the whole cell has a more stable performance and decreased energy consumption is required. However, for aluminum electrolysis the theoretical cell voltage using a non-consumable anode is 2.2 V, which is about 1 V higher than that of a consumable carbon anode. Inert anodes with various materials (mostly NiFe2O4 and SnO2-based materials) have been investigated extensively in laboratory and pilot cells, but none of them have so far been successfully applied in aluminum electrolysis.
Gas anodes, which combine the advantages of carbon and inert anodes, have attracted more and more attention. Early in the 1960s, porous carbon was mainly used as the anode and a small depolarization effect was observed in molten cryolite for aluminum production [2-4]. In a US patent in 2000, a non-consumable anode of the type used for solid oxide fuel cells (SOFC) supplied with reformed natural gas was made for the aluminum electrolysis [5]. Later, several papers [6-8] reported the solid oxide membrane technology for producing metals from their oxides in molten salts, with the introduction of hydrogen. In addition, a nickel-based hydrogen diffusion anode was investigated in an experimental study of aluminum electrolysis, showing measurable depolarization of the anode potential in 2011 [9].
Investigations on the gas anodes have not been carried out extensively. The main focus has been on porous carbon anodes and the type of SOFC with a zirconia-based solid membrane. They can be involved into the anode reaction or corrosion by the electrolyte. SnO2-based materials are good candidates due to their low solubility in molten cryolite and good electrical conductivity. The authors have demonstrated the depolarization effects of Pt and SnO2-based gas anodes in molten chlorides [10-13] and in molten cryolite [14]. In this work, hydrogen was introduced into SnO2-based gas anodes during aluminum electrolysis to demonstrate the involvement of hydrogen in the anode reaction with the overall reaction shown below:
Al2O3+3H2=2Al+3H2O (1)
The standard potentials for different electrode reactions (vs Al3+/Al) in the system are shown in Table 1. The Gibbs energies for calculating the potentials in Table 1 were obtained by the thermodynamic software program HSC.
Table 1 Standard potentials for different electrode reactions versus aluminum reference electrode in molten cryolite saturated with alumina at 850 °C
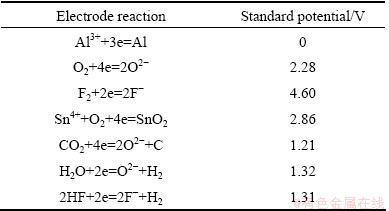
2 Experimental
Stannex ELR blocks (Dyson Thermal Technologies) were used to prepare porous SnO2-based anodes. The green body was a mixture of crushed blocks (Stannex ELR), screened to coarse grains of 180-355 mm, fine 96%SnO2-2%CuO-2%Sb2O3 (mass fraction) powders and CMC as binder, which was rammed inside alumina tubes and then sintered at 1300 °C for 3 h. The fine ceramic powder mixture was used as binder phase during sintering to enhance the mechanical properties. In the designed SnO2-based depolarized gas anode an inner steel tube for feeding gas was installed and surrounded concentrically by an outer steel tube with a bypass valve at the top, as shown in Fig. 1. The gases were introduced from the inner tube, which made the gases pass through the porous anode and into the electrolyte when the valve was closed.
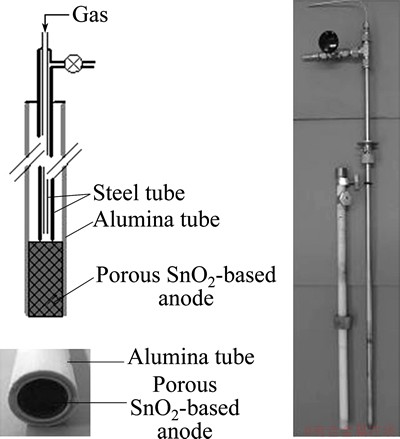
Fig. 1 Schematic diagram of SnO2-based depolarized gas anode
Na3AlF6 (natural cryolite, Greenland) and AlF3 (Noralf, Boliden Odda AS) were mixed at the molar ratio of 1.59, and Al2O3 was added at a mass fraction of 4.5% (Merck, >99%). All the compounds were dried at 200 °C in air for at least 48 h. The system was operated at 850 °C and the electrolyte was saturated with alumina. The bottom of a carbon crucible lined with an alumina tube inside acted as the cathode (counter electrode). Both platinum (d=1 mm) (K. A. Rasmussen, Norway, 99.99%) and SnO2-based materials were used as the working electrode for cyclic voltammetry and the latter was used as the anode for aluminum electrolysis. An Al3+/Al electrode was applied as the reference electrode. All the potentials in molten cryolite were referred to the aluminum reference electrode. The schematic of the electrochemical cell was described in Ref. [14].
The electrochemical measurements of the system were carried out by an Autolab potentiostat (PGSTAT 30) operating with GPES software (General purpose electrochemical system), with an upper limit of 1 A.
3 Results and discussion
Cyclic voltammetry was carried out using platinum and SnO2-based working electrodes in molten Na3AlF6-AlF3-Al2O3 at 850 °C. Platinum is a good material to be used to study the oxygen evolution in molten cryolite [15]. The cyclic voltammograms are shown in Fig. 2. On platinum wire the potential scan started from 1.75 to 2.4 V and then swept back. The reverse scan almost coincided with the initial one. At potentials negative to 2.3 V, the current was almost zero, indicating no occurrence of electrochemical reactions. From 2.3 V, the anodic current began to increase sharply. This value is close to the theoretical standard potential for oxygen evolution listed in Table 1, indicating that the large anodic current corresponds to oxygen evolution. XIAO [15] observed oxygen evolution at ~2.2 V with respect to aluminum in molten cryolite saturated with alumina at 1030 °C. Hence, oxygen evolution occurred at ~2.3 V in this system according to Reaction (2). In the molten electrolytes prepared in this work, O2- represents the complex anions containing oxygen, e.g., Al2OF62- and Al2O2F42- [1]. The simplified anode reaction can be written as follows:
2O2-=O2+4e (2)
During the initial scan on the dense SnO2-based anode, there was almost no current before reaching 2.25 V. Then, the current increased until 2.8 V, which was the upper limit. Before oxygen evolution, expected to occur at 2.28 V theoretically according to Table 1, some residual current was observed. It might be due to the reduction oxidation reactions involving corrosion products from the SnO2-based anode [15] since there was no residual current when platinum was used as the working electrode.
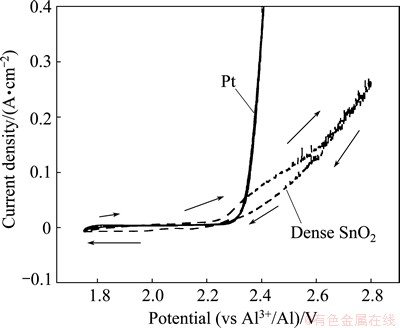
Fig. 2 Cyclic voltammograms on Pt wire and dense SnO2-based anodes in molten Na3AlF6-AlF3-Al2O3 (4.5%) at 850 °C and scan rate of 10 mV/s
From the above results, the anode potential was controlled to be above 2.3 V (e.g., 2.4-3 V) in the constant current electrolysis while no gas was introduced to enable the occurrence of Reaction (2). Then, the designed SnO2-based depolarized gas anode was tested with the introduction of hydrogen. Galvanostatic electrolysis was carried out in molten Na3AlF6-AlF3- Al2O3 (4.5%) at 850 °C. Figure 3 shows the anode potential and cell voltage as function of time at an apparent current density of 0.1 A/cm2. For 03/min) was supplied, an instant decrease occurred and then the anode potential became stable. For 110 min<T<280 min argon was replaced by hydrogen. After 10 min, the anode potential decreased to 1.9 V gradually. At the same time the cell voltage went down to around 1 V. The lower levels for the anode potential and the cell voltage were maintained for around 40 min before increasing to higher values without changing the gas supply.
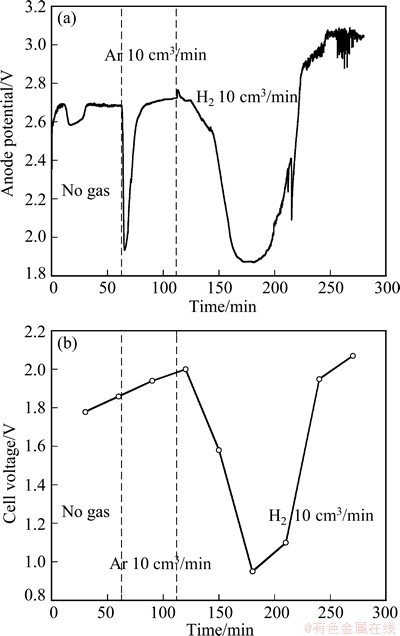
Fig. 3 Anode potential (a) and cell voltage (b) vs time during constant current electrolysis (0.1 A, 0.1 A/cm2) using SnO2- based depolarized gas anode in molten Na3AlF6-AlF3-Al2O3 (4.5%) at 850 °C
During the first 120 min, oxygen was formed according to Reaction (2). The instant decrease caused by the introduction of argon was likely due to the agitation. It was evident that the introduction of hydrogen led to a significant decrease of the anode potential. Compared to the introduction of argon, it was concluded that hydrogen was involved in the anode reaction, resulting in its depolarization effect according to Reaction (3).
O2-+H2=H2O+2e (3)
The depolarization effect of hydrogen was observed with a decrease of anode potential and cell voltage by 0.8 V and 1 V, respectively, although the lower potential could not be maintained for a long time. It is not clear why the lower potential could not be maintained for a long time. It might be due to insufficient supply of O2- or some barrier of hydrogen transfer to the three-phase boundary after some time, which needs to be further explored. In addition, some delay, of about 10 min, was observed from the introduction of hydrogen to a change in the anode potential, due to the fact that the distance from the gas reservoir to the anode is approximately 10 min at a flow of 10 cm3/min.
The theoretical consumption of hydrogen during the constant current electrolysis at a current of 0.1 A was calculated as 2.9 cm3/min according to Reaction (3). In this electrolysis, the amount of supplied hydrogen is adequate for the complete occurrence of Reaction (3) theoretically.
From Table 1 the theoretical anode potential difference between Reactions (2) and (3) is around 1 V, which is consistent with the measured value, indicating that the electrochemically active sites for the three-phase-boundary Reaction (3) were successfully built. The three phases include O2- in molten cryolite, electrons from the solid anode and reducing gas from the gas phase.
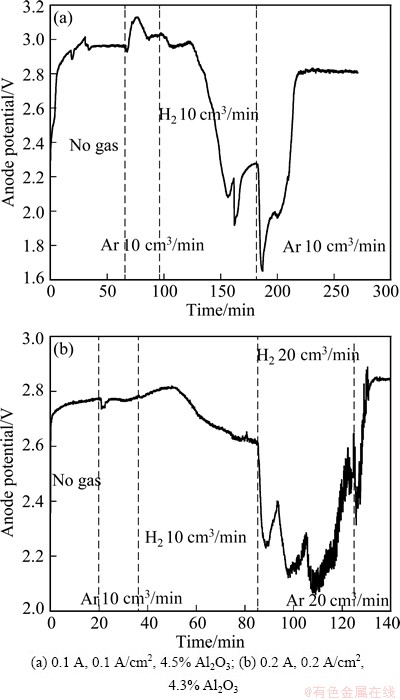
Fig. 4 Anode potentials vs time during constant current electrolysis under different conditions using SnO2-based depolarized gas anodes in molten Na3AlF6-AlF3-Al2O3 at 850 °C
At least two experiments were carried out to confirm the reproducibility of the depolarization effect of the reducing gas for aluminum electrolysis. The results are shown in Fig. 4. Figure 4(a) shows that after the introduction of hydrogen (10 cm3/min) the anode potential was found to decrease by around 0.8 V. In Fig. 4(b) the gas anode was also tested at a larger apparent current density of 0.2 A/cm2. After the introduction of hydrogen with a flow rate of 10 cm3/min, the anode potential was decreased by 0.2 V. By increasing the hydrogen flow to 20 cm3/min, a further decrease of 0.4 V was observed. In this case the application of a larger current resulted in more hydrogen (20 cm3/min) needed to achieve an obvious depolarization effect.
4 Conclusions
1) In molten cryolite saturated with alumina, oxygen evolution on the platinum and the SnO2-based anode was investigated and a residual current before the release of oxygen on the SnO2-based anode was observed.
2) The depolarization effect of hydrogen was demonstrated during constant electrolysis at an apparent current density of 0.1-0.2 A/cm2 on the designed SnO2-based depolarized gas anodes. The decrease of the anode potential and cell voltage reach 0.8 and 1 V, respectively, which are close to the theoretical values.
Acknowledgments
The authors are grateful for the financial support from the GASSMAKS program of the Norwegian Research Council, Grant No. 182546/I30 and for valuable discussion with staff from SINTEF and Department of Materials Science and Engineering, Norwegian University of Science and Technology.
References
[1] THONSTAD J, FELLNER P, HAARBERG G M, HIVES J, KVANDE H, STERTEN A. Aluminium electrolysis: Fundamentals of the Hall-Héroult process [M].
: Aluminium-Verlag Marketing & Kommunikation GmbH, 2001: 1-4.
[2] FERRAND M L. Note dy M. Louis ferrand [J]. Bulletin de la Societe Francaise Des Electriciens, 1957, 79: 412-413. (in French)
[3] STENDER V V, TROFIMENKO V V. One solution to the anode problem in the electrolytic production of aluminum [J]. Khim Tekhnol, 1969, 12: 41-45.
[4] KRONENBERG M L. Gas depolarized graphite anodes for aluminum electrowinning [J]. J Electrochem Soc, 1969, 116: 1160-1164.
[5] RAPP R A. Method featuring a non-consumable anode for electrowinning of aluminium: US6039862 [P]. 2000-03-21.
[6] PAL U B, WOOLLEY D E, KENNEY G B. Emerging SOM technology for the green synthesis of metals from oxides [J]. JOM, 2001, 53: 32-35.
[7] KRISHNAN A, LU X G, PAL U B. Solid oxide membrane process for magnesium production directly from magnesium oxide [J]. Metall Mater Trans B, 2005, 36: 463-473.
[8] PAL U B, POWELL IV A C. The use of solid-oxide-membrane technology for electrometallurgy [J]. JOM, 2007, 59: 44-49.
[9] NAMBOOTHIRI S, TAYLOR M P, CHEN J J, HYLAND M M, COOKSEY M A. An experimental study of aluminum electrowinning using a nickel-based hydrogen diffusion anode [J]. Electrochimica Acta, 2011, 56: 3192-3202.
[10] XIAO S J, MOKKELBOST T, HAARBERG G M, RATVIK A P, ZHU H M. Depolarized gas anodes for electrowinning of metals in molten salts [J]. Electrochemical Society Transactions, 2010, 28: 361-366.
[11] MOKKELBOST T, PAULSEN O, XIAO S J, HAARBERG G M, RATVIK A P. Fabrication and properties of SnO2-based inert gas anodes for electrowinning [J]. Electrochemical Society Transactions, 2010, 28: 211-219.
[12] HAARBERG G M, KVALHEIM E, RATVIK A P, XIAO S, MOKKELBOST T. Depolarised gas anodes for aluminium electrowinning [J]. Transactions of Nonferrous Metals Society of China, 2010, 20: 2152-2154.
[13] XIAO S J, HAARBERG G M. Depolarized SnO2-based gas anodes for electrowinning of silver in molten chlorides [J]. Journal of Mining and Metallurgy, 2013, 49(1): 71-76.
[14] XIAO S J, MOKKELBOST T, PAULSEN O, RATVIK A P, HAARBERG G M. SnO2-based gas (methane) anodes for electrowinning of aluminum [J]. Metall Mater Trans B, 2013, 44: 1311-1316.
[15] XIAO H M. On the corrosion and the behavior of inert anodes in aluminium electrolysis [D]. Trondheim: Norwegian Institute of Technology, 1993: 30-40.
铝电解中二氧化锡基(氢气)气体阳极
肖赛君1,2, Tommy MOKKELBOST3, Ove PAULSEN3, Arne P. RATVIK2, Geir M. HAARBERG2
1. 安徽工业大学 冶金工程学院,马鞍山 243002;
2. Department of Materials Science and Engineering, Norwegian University of Science and Technology, Trondheim 7491, Norway;
3. SINTEF Materials and Chemistry, Trondheim 7465, Norway
摘 要:开发一种在低温电解质(850 °C)铝电解中应用的新型二氧化锡基气体阳极以降低铝电解能耗和二氧化碳排放。在此种气体阳极中,氢气通入阳极后参与阳极反应,分别用石墨和铝作阴极和参比电极。采用循环伏安法研究此体系中铂和二氧化锡基电极上氧离子的电化学行为,并确定氧气的析出电势。然后,采用气体电极进行恒电流电解实验,相对于未通入气体和通入氩气,通入氢气后阳极出现明显的去极化现象(阳极电势约下降0.8 V)。实验结果表明,氢气已参与阳极三相界面(气体-电解质-电极)反应,为还原性气体阳极在铝电解上的应用提供了可行性。
关键词:二氧化锡基气体阳极;氢气阳极;铝电解
(Edited by Wei-ping CHEN)
Foundation item: Project (51404001) supported by the National Natural Science Foundation of China; Project ([2014]1685) supported by the Scientific Research Foundation for the Returned Overseas Chinese Scholars, Ministry of Education, China
Corresponding author: Sai-jun XIAO; Tel: +86-18255576998; E-mail: jxddroc@126.com
DOI: 10.1016/S1003-6326(14)63551-2