
Lead electrodeposition from alkaline solutions containing xylitol
GU Ying-ying1, 2, ZHOU Qiong-hua1, YANG Tian-zu3, LIU Wei3, ZHANG Du-chao3
1. School of Chemistry and Chemical Engineering, Central South University, Changsha 410083, China;
2. Key Laboratory of Resources Chemistry of Nonferrous, Central South University, Changsha 410083, China;
3. School of Metallurgical Science and Engineering, Central South University, Changsha 410083 , China
Received 25 June 2010; accepted 12 October 2010
Abstract: The electrodeposition of lead in alkaline solutions containing xylitol (1, 2, 3, 4, 5-pentahydroxypentane) was studied. The lead electrodeposition and the chemical stability of xylitol in alkaline solutions were investigated by cyclic voltammetry. Apparent activation energy, apparent transfer coefficient and exchange current density were obtained by linear sweep voltammetry. Initial stages of lead electrocrystallization were determined by chronoamperometry. Voltammograms of a AISI 316 stainless steel electrode in xylitol solution exhibit no current in the potential range of -1.3?V to 0.75?V (vs Hg/HgO), implying that xylitol is stable to oxidation and reduction. The apparent activation energy, apparent transfer coefficient and exchange current density were calculated to be 35.15?kJ/mol, 1.56 and 9.65×10-5?A/m2. Analysis of the chronoamperometric responses implies three-dimensional growth of nuclei, with the type of nucleation depending on overpotential.
Key words: lead; xylitol; electrodeposition; cyclic voltammetry; nucleation; chronoamperometry
1 Introduction
Lead is refined by both pyrometallurgical and hydrometallurgical processes. The hydrometallurgical process is widely applied in industry due to a higher recovery rate of precious metals and low energy consumption. The electrolytes in lead electrodeposition are usually acidic and based on fluorosilicate, chloride [1], bromide, iodide [2], nitrate [3] and fluoborate [4]. The process for electrorefining of lead most applied industrially is the Betts process, which uses a fluorosilicate electrolyte containing PbSiF6 and H2SiF6 [5]. The process ensures effective enrichment of rare and noble metals in the anode slime and the deposition of high-purity grade lead. Nevertheless, the fluorosilicate electrolytes are usually volatile and decompose to toxic HF and SiF4, which cause air pollution.
Alkaline electrolytes are assumed to be less harmful to human heath and more appropriate from an environment standpoint [6]. However, to our knowledge, only a few reports have been published in this field. WONG and ABRANTES [7] used chronoamperometry to study lead electrodeposition from alkaline solutions, and found that the lead (II) concentration affected the type of nucleation. Recently, it was reported that antimony oxide and lead oxide could be dissolved in alkaline solutions containing polyhydric organic compounds [8], such as C5H12O5 and C3H8O3. Such compounds may offer an alternative for electrowinning or electrorefining of metals. ZHANG et al [9] reported a new process and optimization of the process parameters for separating gold from antimony-gold alloys in alkaline solution containing xylitol. Publications on alkaline electrolysis of lead have not been focused on electrorefining, but on electrowinning from secondary sources of lead, such as end-of-life lead-acid batteries. MORACHEVSKII et al [10] first reported the feasibility of separating lead from exhausted lead-acid batteries in alkaline glycerol media. CARLOS et al [11] investigated lead electrodeposition on copper from alkaline glycerol solutions, and concluded that the lead film on the copper substrate was strongly adhered and EDS showed the presence of a lead film on the Cu substrate from the initial stage of the deposition process. Compared with acidic medium, alkaline solution electrolysis is a better alternative because of low corrosion, less toxicity, and less volatility. In addition, as a food addictive, the xylitol is poisonless and harmless [12-13]. The electrowinning and electrorefining of lead with alkaline solutions containing xylitol will reduce environment pollution.
However, there are few reports in the literature on the electrochemical behavior of lead electrodeposition from alkaline media. The electrodeposition mechanism and types of nucleation at the initial stages of the lead deposition process are not well established in reports related to alkaline media containing xylitol.
In this work, the electrodeposition of lead from alkaline media containing xylitol was investigated by cyclic voltammetry (CA), linear sweep voltammetry (LSV) and chronoamperometry (CA). The mechanism of lead electrodeposition and types of nucleation were discussed. The diffusion coefficient of lead was also determined. The aim of this work is to provide better understanding of the underlying mechanisms in the lead electrorefining process.
2 Experimental
A CHI 660C electrochemical analyzer (CH Instrument) was employed to do the electrochemical measurements. A glass three-electrode electrochemical cell was used with a AISI 316 stainless steel (SS316) working electrode of 1?cm2, one side of which was masked with an insulating lacquer made of epoxide resin and diamine. The counter electrode was a platinum flag and the reference electrode was a Hg/HgO electrode (Shanghai Shenyuan Science Instrument Co. Ltd.), with standard electrode potential of 0.114?V (vs. SHE) at 298?K, against which all electrode potentials are reported in this work. Before every electrochemical measurement, the electrodes were polished with metallographic SiC sand paper. Subsequently, the electrodes were polished with alumina powders down to 0.05?μm in size and then cleaned with deionized water in an ultrasonic bath. All the measurements were carried out at 298 K unless otherwise specified.
The xylitol used in experiments was of food grade (purity 99.5%), produced in Dalian Xigang Yikang Xylitol Food Co. The PbO and the sodium hydroxide were of analytical reagent grade. All the solutions were prepared with deionized water. The solution composition used in this work was obtained from the study on the dissolving ability of lead oxide in the alkaline solution containing xylitol. The composition of electrolyte used in electrochemical stability tests of xylitol in alkaline media was 2.5?mol/L NaOH, 0.6? mol/L C5H12O5. Solution components in other experiments were 2.5 mol/L NaOH, 0.5? mol/L Pb(II) and 0.6?mol/L C5H12O5.
3 Results and discussion
3.1 Electrochemical stability of xylitol in alkaline solutions
During electrolysis, in addition to the electrodeposition of lead, the organic groups may undergo some redox reactions which will affect the stability of the electrolyte composition, current efficiencies, deposit morphology and electrolysis costs. Hence, the electrochemical stability of xylitol in alkaline solutions was studied, in order to identify a potential range in which xylitol undergoes no redox reactions.
Figure 1 shows the cyclic voltammograms of stainless steel and lead electrodes which were made of high pure lead in an alkaline solution containing xylitol at 298 K and 323?K; the initial negative-going potential scan started from the open circuit potential of ca. -0.8?V at a sweep rate of 10?mV/s. Figure 1 (a) shows that currents density increased sharply at potentials < -1.45?V and bubbles appeared on the electrode surface. The value of the cathodic current density increased rapidly as the potential shifted towards more negative values, then reached a maximum and plateau value at E < -1.60?V. On the subsequent positive-going potential scanning, the current density returned to zero at about -1.30?V, at which no hydrogen evolution was evident on the electrode surface. The magnitude of the current density remained essentially zero between -1.30 and 0.6?V, indicating that the electrolyte was stable in this potential range. When E > 0.6?V, oxidation currents began to flow and bubble, presumably oxygen was observed on the electrode surface. The anodic current density increased at more positive potentials and reached its maximum at 0.8?V. Increasing the electrolyte temperature from 298?K to 323?K had little effect on the potential of hydrogen evolution.
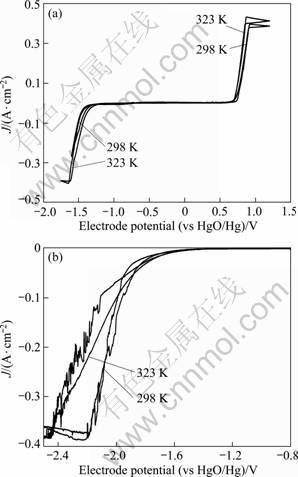
Fig. 1 Cyclic voltammograms of stainless steel electrode (a) and lead electrode (b) in alkaline solution containing xylitol
Comparison of Figs. 1(a) and (b) shows that, as expected, the overpotential for hydrogen evolution on the lead electrode was higher than that on the stainless steel electrode. At 298 K, the hydrogen evolution reaction occurred at E < -1.6?V, below which reduction current density increased rapidly. At potentials <-2.1?V, some small current density peaks appeared which may indicate decomposition of xylitol at the cathode, though no reports have been published about the mechanism of xylitol reduction reactions at cathodes. The maximum current density of hydrogen evolution reaction appeared at about -2.45?V, which was close to that of the stainless steel electrode. At 323?K, the potential of hydrogen evolution reaction did not increase for the lead electrode, but at more negative potentials, the cathodic current density increased more rapidly, and then reached a maximum current density at about -2.2?V. Compared with the limiting current density at 298?K, it shifted by about 0.25?V to less negative potentials. When E < -2.0?V, there was also some small current density peaks, probably associated with the growth and departure of hydrogen bubbles changing the effective cathode area.
3.2 Cyclic voltammogram of lead deposition
Figure 2 shows a typical cyclic voltammogram of lead deposition in alkaline solution containing xylitol. During the negative-going potential scanning, the cathodic current density increased sharply at potentials < -0.79?V as a result of lead deposition, leading to a current density peak at about -0.82?V. On the positive-going potential sweep, lead dissolution resulted in the current density peak at -0.6?V. Lead electrodeposition occurred within the range of -1.30 to 0.5?V, in which the xylitol in the electrolyte was predicted to be stable from the results in the previous section.
Figure 3 shows the effect of potential sweep rate on cyclic voltammograms of stainless steel in the solution containing 2.5? mol/L NaOH, 0.5? mol/L Pb(II) and 0.6? mol/L xylitol (C5H12O5). The negative-going potential sweep was initiated from the open circuit potential of -0.72?V, resulting in only one reduction peak, at -0.82?V, assigned to lead electrodeposition, before hydrogen evolution at more negative potentials. The maximum current density value increased more sharply with higher sweep speed while the potential of the peak moved to more negative values. The shape of all the cyclic voltammograms remained almost the same with increasing the sweep speed, and the potential of the peak shifted towards negative direction.
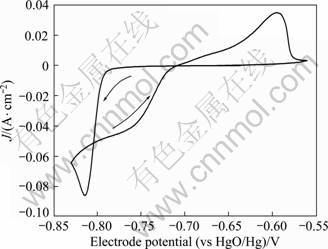
Fig. 2 Cyclic voltammogram of AISI 316 stainless steel of 1 cm2 in solution containing 2.5 mol/L NaOH, 0.5 mol/L Pb(II) and 0.6 mol/L xylitol (C5H12O5) at sweep rate of 10 mV/s
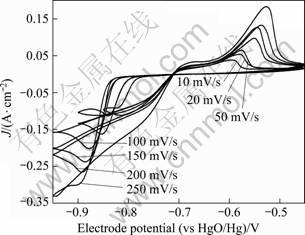
Fig. 3 Effect of potential sweep rate on cyclic voltammograms of AISI 316 stainless steel of 1?cm2 in solution containing 2.5? mol/L NaOH, 0.5? mol/L Pb(II) and 0.6? mol/L xylitol (C5H12O5)
3.3 Determination of kinetic parameters of lead deposition
3.3.1 Apparent activation energy
The value of the activation energy for an electrode reaction is an important parameter in the determination of the reaction mechanism [14]. Figure 4 shows the cathodic polarization curves of lead electrodeposition in alkaline solution containing xylitol at different temperatures. As can be seen from Fig. 4, with increasing the temperature, the lead deposition potential shifted to the positive values gradually, suggesting that higher temperature could enhance the electrodeposition velocity of lead. Moreover, as the temperature increased, the maximum current density value of lead deposition increased significantly. This phenomenon indicated that at the same overpotential, there would be a larger discharge current density at higher temperature. Taking an overpotential of -0.035?V and the current density at different temperatures, the relationship between exchange current density and T -1 is shown in Fig. 5. The linear fitting equation is
(1)
and the formula for the calculation of apparent activation energy is
(2)
where B is constant; A is apparent activation energy; J is the current density; T is temperature. The calculated apparent activation energy is 35.15?kJ/mol.
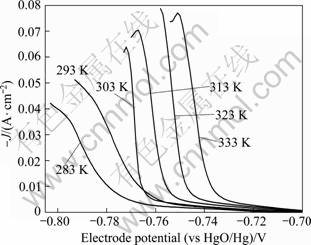
Fig. 4 Cathode linear sweep voltammograms of Pb electrodeposition on AISI316 stainless steel in alkaline solution containing xylitol at different temperatures and scan rate of 1 mV/s
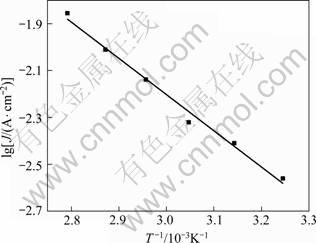
Fig. 5 Relationship between exchange current density and T -1 in alkaline solution containing xylitol
3.3.2 Apparent transfer coefficient
Figure 6 shows the steady-state polarization curves of lead electrodeposition. The Tafel equation obtained by the linear fitting, from -0.055?V to -0.065?V, is as follows:
(3)
According to the following formula:
(4)
where αn is the apparent transfer coefficient; F is Faraday’s constant; ηc is cathode overpotential; J0 is exchange current density; Jc is cathodic current density.
Transforming the cathodic polarization curve (Fig. 6) into η-lg Jc curve (in Figure 7), by linear fitting, the kinetic parameters αn and J0 were calculated to be 1.56 and 9.65×10-5?A/cm2, respectively. Compared with the data in other reports [15], the smaller J0 in this work indicated that lead electrodeposition required larger driving forces in this particular alkaline solution.
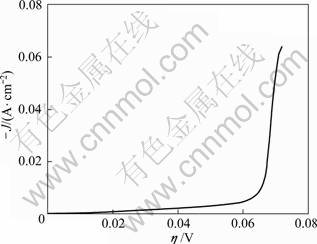
Fig. 6 Steady-state polarization curves for lead deposition on AISI316 stainless steel
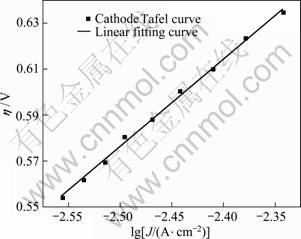
Fig. 7 Tafel plot for lead deposition
3.4 Chronoamperometry for nucleation modeling
It is can be seen from Fig. 2, the cathodic current density appeared at the potential of -0.75?V which was associated with electrodeposition of lead. At more negative potentials, the current density increased rapidly, and there was a clear reduction peak at -0.82?V. During the reverse sweep, two crossovers between cathodic current density branches were observed, suggesting the occurrence of metal electrocrystallization on the cathode.
Figure 8 shows a family of potentiostatic current transients of lead electrodeposition in alkaline solution containing xylitol. The current transients consist of an initial fast current decay attributing to double-layer charging which is significant for t < 1 s [15]. This is shown in Fig. 8(a) (near the Y axis), where the transient current falls from a higher starting current. This is because, in the initial stage of the lead crystallization, the lead atoms first aggregate to form atom clusters and the critical nuclei are formed. The transients were characterized by, firstly, a rapid growth of current up to a maximum Im at time tm, and then followed by a slow decay. The increase of cathodic current was due to the increase of the electroactive area, which included the growth of each independent nucleus and the number of nuclei. The descending current after this stage related to linear electrode diffusion and the declining current followed Cottrell’s equation. So, the lead deposition consisted of nucleation, overlapping of nuclei, elimination of growth centers and initiation of new growth centers [16]. It also can be found in Fig. 8 that, as the potential was set more negative, Im increased and tm decreased, which is usually found for the nucleation of solid phases produced electrochemically.
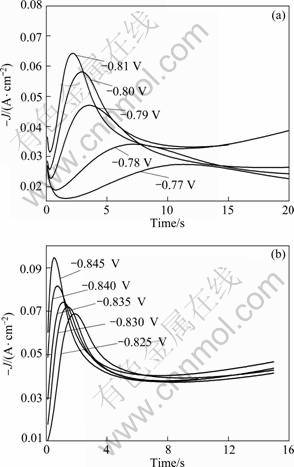
Fig. 8 Potentiostatic current transients of lead electrodeposition
It has been reported that potentiostatic transients could be evaluated through mathematic modeling. SCHARIFKER and HILLS [16] developed a model for three-dimensional (3D) multiple nucleation with diffusion-controlled growth. According to the assumptions of random distribution of the hemisphere- shaped nuclei, no new nuclei occurred in the growth region of every existed nucleus. The overlapping of the growth zones, the expressions of instantaneous and progressive nucleation with 3D growth are presented in Table 1. In Table 1, zF is molar charge for the deposition; D is diffusion coefficient; c is ion concentration; N0 is nucleation density; A is nucleation rate constant; k and k′ are reaction rate constants.
Figure 9 shows the normalized cathodic transients from Fig. 8 together with the theoretical curves which were evaluated from equations in Table 1 for instantaneous and progressive 3D nucleation and growth. Curves with different shapes were obtained due to different nucleation mechanisms. The peak of instantaneous plots is sharp while the progressive is relatively gentle. As illustrated in Fig. 9, when overpotential was relatively small (-0.77 to -0.835?V), the experimental data followed progressive nucleation. When E < -0.835?V, there was a rise in cathode overpotential and a tendency towards instantaneous nucleation was revealed. Progressive nucleation may be due to a smaller Pb nucleus density during a very short time for double-charging at low overpotential. These nuclei continued to precipitate in the electrocrystallization process. However, at high overpotential, a large number of nuclei were generated during the short time for double-charging. Electrochemical crystallization is mainly the growth of these nuclei, which followed the ‘instantaneous nucleation’.
Table 1 Formula of nucleation mechanism
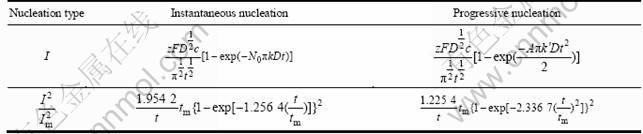
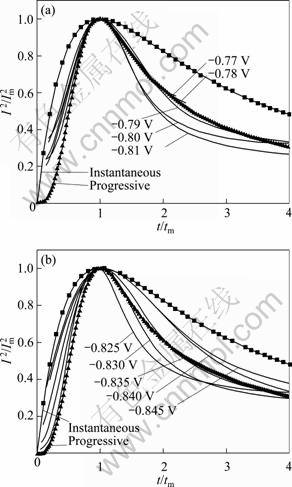
Fig. 9 (I/Im) 2 vs t/tm plots of experimental data of potentiostatic for Pb electrodeposition
According to the equation of maximum transient current:
Im=zFK′ (5)
where K′ is the crystal growth rate vertical to the crystal surface, which can be evaluated. The plot of lg K′ against imposed potentials is shown in Fig. 10. It can be seen, when E > -0.81?V, the plot exhibited a linear relationship which indicated that potential has a significant influence on the crystal growth rate, and the crystal growth rate rose as the applied potential shifted negatively. When E < -0.81?V, the crystal growth rate was close to a limiting value on which the applied potential has little effect.
According to the following equation:
(6)
Substituting the I2mtm value at different potentials into Eq. (6), the value of diffusion coefficient could be calculated (illustrated in Table 2). The value of diffusion coefficient changed little as the applied potential shifted and the average value D was 3.65×10-6?cm2/s.
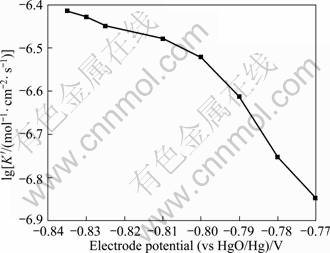
Fig. 10 Effect of potential on nucleation growth rate coefficient
Table 2 Analysis of transients of lead deposition in Fig. 8
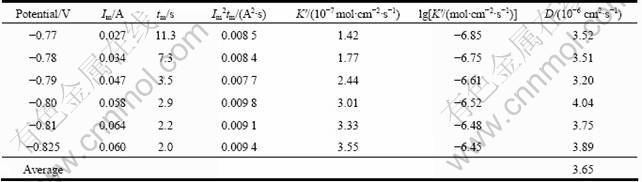
4 Conclusions
1) Cyclic voltammograms indicate that xylitol exhibits good electrochemical stability in alkaline solutions and that no chemical reactions occur within the potential range of -1.30?V to 0.6?V. In the range between -1.75 and 1.25?V, there is no other chemical reaction but water electrolysis reactions. While at the potential < -2.0?V, xylitol may be decomposed in the electrode.
2) Cyclic voltammograms in alkaline solution containing xylitol at different sweep rates show that the lead electrodeposition process involves nucleation on AISI316 stainless steel. According to the Tafel plot, the apparent transfer coefficient and exchange current density were calculated to be 1.56, and 9.65×10-5?A/m-2, respectively.
3) Analysis of the chronoamperometric responses support the view of a three-dimensional growth and suggest a substantial influence of overpotential on the type of nucleation. The electrocrystallization of lead follows progressive nucleation during the potential region between -0.77?V and -0.835?V and instantaneous nucleation when the potential is more negative than -0.835?V. The average diffusion coefficient of lead was calculated to be 3.65×10-6?cm2/s.
References
[1] DOULAKAS L, NOVY K, STUCKI S, COMNINELLIS C H. Recovery of Cu, Pb, Cd and Zn from synthetic mixture by selective electrodeposition in chloride solution [J]. Electrochimia Acta, 2000, 46(3): 349-356.
[2] MOSTANY J, PARRA J, SCHARIFKER B R. The nucleation of lead from halide-containing solutions [J]. Journal of Applied Electrochemistry, 1986, 16: 333-338.
[3] AVELLANEDA C O, NAPOLITANO M A, KAIBARA E K. Electrodeposition of lead on ITO electrode: Influence of copper as an additive [J]. Electrochimia Acta, 2005, 50(6): 1317-1322.
[4] EXP?SITO E, GONZ?LEZ-GARC?A J, BONETE P. Lead electrowinning in a fluoborate medium [J]. Journal of Power Sources, 2000, 87: 137-142.
[5] DOBREV T, RASHKOV S. Processes during the electrorefining and electrowinning of lead[J]. Hydrometallurgy, 1996, 40(3): 277-291.
[6] CARLOS I A, SIQUEIRA J L P, FINAZZI G A. Voltammetric study of lead electrodeposition in the presence of sorbitol and morphological characterization [J]. Journal of Power Sources, 2003, 117: 179-184.
[7] WONG S M, ABRANTES L M. Lead electrodeposition from very alkaline media [J]. Electrochimica Acta, 2005, 51(4): 619-626.
[8] LIU Wei, YANG Tian-zu, ZHANG Du-chao, XIA Xin, LIU Wei-feng. Performance on leaching of antimony trioxide with polyhydric organics in alkaline solutions. TMS/2009[C]//Supplemental Proceedings. San Francisco, CA, USA, 2009: 403-411.
[9] ZHANG Du-chao, YANG Tian-zu, LIU Wei, LIU Wei-feng, XIE Zhao-feng. Electrorefining of a gold-bearing antimony alloy in alkaline xylitol solution[J]. Hydrometallurgy, 2009, 99: 151-156.
[10] MORACHEVSKII A G, DEMIDOV A I, VAISGANT Z I. Recovery of lead battery scrap using alkali-glycerol electrolyte [J]. Russian Journal of Applied Chemistry, 1996, 69(3): 412-414.
[11] CARLOS I A, MATSUO T T, SIQUEIRA J L P, DE ALMEIDA M R H. Voltammetric and morphological study of lead electrodeposition on copper substrate for application of a lead-acid batteries [J]. Journal of Power Sources, 2004, 132: 261-265.
[12] DURAIRAJ L, LAUNSPACH J, WATT J L, MOHAMAD Z, KLINE J, ZABNER J. Safety assessment of inhaled xylitol in subjects with cystic fibrosis [J]. Journal of Cystic Fibrosis, 2007, 6(1): 31-34.
[13] de FAVERI D, TORRE P, PEREGO P, CONVERTI A. Optimization of xylitol recovery by crystallization from synthetic solutions using response surface methodology [J]. Journal of Food Engineering, 2004, 61(3): 407-412.
[14] JIANG Han-ying. Metallurgical electrochemistry [M]. Beijing: Metallurgical Industry Press, 1983: 142-142. (in Chinese)
[15] LIU Yong-hui. The measurement technology of electrochemistry [M]. Beijing: Beijing University of Aeronautics and Astronautics Press, 1987: 126-126. (in Chinese)
[16] SCHARIFKER B, HILLS G. Theoretical and experimental studies of multiple nucleation [J]. Electrochimia Acta, 1983, 28(7): 879-889.
铅在碱性木糖醇体系中的电沉积行为
古映莹1, 2, 周琼华1, 杨天足3, 刘 伟3, 张杜超3
1. 中南大学 化学化工学院,长沙 410083;2. 中南大学 有色金属资源化学教育部重点实验室,长沙 471003;
3. 中南大学 冶金科学与工程学院,长沙 410083
摘 要:探讨铅在碱性木糖醇体系中的电沉积行为;采用循环伏安法研究碱性木糖醇体系的电化学稳定性以及铅在阴极的电沉积过程;利用线性扫描伏安法求解体系的表观活化能、表观传递系数和交换电流密度;采用计时电流法判断铅在电极上的成核方式。结果表明:在扫描电压范围-1.3?~ 0.75?V(vs Hg/HgO)内,木糖醇在碱性溶液中无氧化还原反应;由线性扫描伏安法求得表观活化能为35.15?kJ/mol,表观传递系数为1.56,交换电流密度为9.65×10-5?A/cm2;铅的成核方式遵循三维成核,并取决于过电位。
关键词:铅;木糖醇;电沉积;循环伏安;成核;计时电流法
(Edited by LI Xiang-qun)
Foundation item: Project (2007CB613604) supported by the National Basic Research Program of China
Corresponding author: GU Ying-ying; Tel: +86-731-88879616; E-mail: guyy02@163. com
DOI: 10.1016/S1003-6326(11)60874-1