
Compression behavior of magnesium-lithium alloy at room temperature
WANG Cong (王 聪) 1, LI Zhuo-qun (李卓群) 1, XU Yong-bo (徐永波) 2, HAN En-hou (韩恩厚) 1
1. Environmental Corrosion Center, Institute of Metal Research, Chinese Academy of Sciences,
Shenyang 110016, China;
2. Shenyang National Laboratory for Materials Science, Institute of Metal Research,
Chinese Academy of Sciences, Shenyang 110016, China
Received 28 July 2006; accepted 15 September 2006
Abstract: The mechanical properties of LA40 magnesium alloy were investigated under compression at room temperature. In the applied strain rate range from 1.33×10-4 s-1 to 6.66×10-4 s-1, this alloy shows positive strain rate sensitivity (SRS). Most importantly, the Portevin-Le Chatelier(PLC) effect which is an interesting and dominant feature in tensile tests disappears under compressive conditions. Only one twinning system is activated under tensile deformation, while at least two twinning modes are operative during compression. Additionally, the effect of dynamic strain aging (DSA), and the main mechanism for the onset of PLC phenomenon during tension, will be weakened because the strengthened obstructing influence of twins on solute atoms and the disappearance of PLC is inevitable.
Key words: compression; LA40 magnesium alloy; twinning; PLC effect
1 Introduction
During the past decade, due to the soaring demand of light structural materials, research activities on magnesium alloys have been tremendously conducted world-widely. Despite magnesium alloys’ disadvantages compared with steel or titanium alloys, they possess excellent specific strength and modulus. Among developed magnesium alloy systems, magnesium-lithium based alloys can offer promising properties because of their lowest densities and relatively good workability. Therefore, magnesium-lithium alloys are attractive for structural or secondary structural applications for aerospace, missile and armor applications[1-15].
At present, the mechanical characterizations of magnesium-lithium alloys are mainly concentrated on tensile aspects, leaving compressive properties behind[16-18]. However, according to theoretical predictions and calculations, the deformation mechanisms are more complex when they are subjected to compression. Hence, it is necessary to investigate the compressive properties thoroughly.
2 Experimental
The tested alloy in this study, which is nominated as LA40, has the following chemical compositions: Li 4.06% and Mg balance (in mass fraction). The ingots were firstly extruded at 673 K with the extrusion ratio of 8?1. Subsequently, they were subjected to 1 h homo- genization at 623 K. By quenching in air at room temperature, the samples for compression were spark cut. These samples had the following dimensions: d5 mm×10 mm. The compression tests were carried out on an MTS-810 mechno-hydraulic machine under three different initial strain rates: 1.33×10-4, 3.33×10-4 and 6.66×10-4 s-1. Under each strain rate, at least two samples were compressed in order to guarantee the results. Besides, the stress—strain curves were recorded at the most appropriate date-collection speed.
Microstructural details were revealed under an environmental scanning electronic microscope (ESEM, Philips XL30 FEG). The operation voltage was 10 kV. The employed reagent was composed of 3 g picric acid, 10 mL acetic acid, 10mL distilled water and 50 mL ethyl alcohol.
3 Results
The engineering stress-strain curves of LA40 under three different strain rates were recorded to give direct comparison (Fig.1). It is clearly seen that each stress-strain curve can be divided into three distinct parts, that is, linear-elastic region, rapid work-hardening regime as well as parabolic-like slow work-hardening domain. An inflection, occurring around 7% strain, exists in the rapid work-hardening region, indicating more slip and twinning systems are involved after that point.
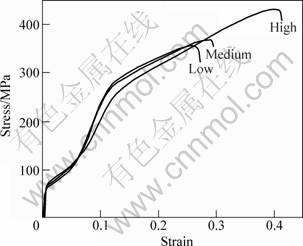
Fig.1 Stress-strain curves of LA40 under different strain rates
Another point that deserves notice is that strain rate will impose significant influence on LA40. With increasing the strain rate (Low, Medium and High in Fig.1, respectively), the yielding stresses are almost identical, but the ultimate compressive stresses are enhanced (from 359.9 MPa through 371.2 MPa to 435.9 MPa). That is to say, LA40 has positive strain rate sensitivity (SRS) under compression conditions. What is more, the plasticity of LA40 undergoes a dramatic increase with elevated strain rate.
Considerations solely focused on compression conditions provide relatively less information, while the comparison with tensile experiments will offer more latitude to delve further into deformation mechanism.
Fig.2 shows the corresponding stress-strain curve of LA40 under the tensile strain rate of 6.66×10-4 s-1. Obviously, the stress-strain curve under tension is quite different from that under compression. The tensile curve lacks the above-mentioned rapid work hardening regime and the inflection; however, it has an almost horizontal stress plateau when high strain is achieved. Though difference between yielding stresses is not large, the ultimate tensile stress(approximate 140 MPa) is far less than corresponding ultimate compressive stress. The overall plasticity remains the same under different stresses. Another point that should be noted is that under compression, the stress-strain curves are smooth through the deformation process, however, when tensile stress is applied, the smooth curve becomes serrated after an incubation strain. As always, the so-called serrations occurring on the stress-strain curves are manifestations of Portevin-Le Chatelier effect (PLC effect). Briefly, the PLC effect, which is an important phenomenon during tension, disappears under compression.
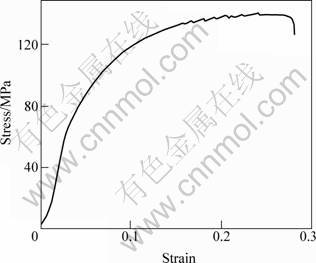
Fig.2 Stress-strain curve of LA40 at tensile strain rate of 6.66×10-4 s-1
4 Discussion
As we know, in HCP alloys, the two most common slip systems, basal and prismatic slips, offer only four independent modes between them[19]. In order to accommodate the inhomogenity imposed by lacking of sufficient slip mode, twinning must be initiated during deformation.
Generally speaking, common twinning modes in HCP alloys are as follows:
,
,
,
, with the former two being tensile category and the latter two compressive ones[19-20]. Since the critical resolved shear stress (CRSS) of
is very high, it cannot be initiated at the beginning of deformation. Therefore, it is believed that
twinning constitutes the only possible twinning mode at the incipient period of compression. According to YOO’s explanations [19], CRSSes for
compression twins and
tension twins are almost identical. With other conditions equal, it can be concluded that their yielding stresses have almost the same values.
As deformation proceeds, more
twins are produced. The intense interaction between dislocations and twins will definitely make further deformation harder, bringing about moderate work-hardening regime. However, after certain amount of strain (about 7%) is achieved,
compression twinning could probably be initiated as a result of the fact that the stress for nucleation of
twins continues to rise until it exceeds the stress for
twinning.
The microstructures of LA40 before deformation and after inflection point are presented in Fig.3. It can be clearly seen that parallel twins are produced after certain amount of deformation. These twins must belong to different modes. They either lie inside a grain or end at grain boundaries (GBs). Consequently, more and more twinning modes are active and their interactions with dislocations are more and more intense, and a rapid work hardening domain is apparent.
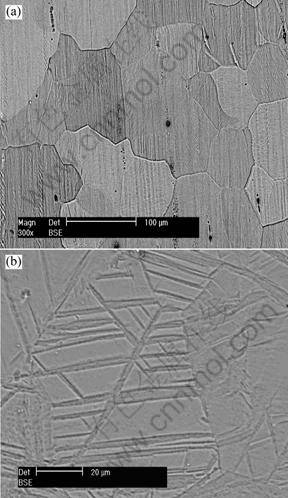
Fig. 3 Microstructures of LA40 before compression (a) and after 8% strain (b)
When large strain is accumulated, interactions between twins and dislocations are reduced. As a result, the work hardening rate should be lowered. Fig.4 reveals the microstructure of LA40 after rapture. Compared with Fig. 3, it can be concluded that more twins are produced. Additionally, some of the twins are fragmented, manifesting that interactions among twins should also be very intense. In other words, both interactions between dislocations and twins and those of twins themselves should be incorporated together to shed some light on the observed phenomena.
That compression induces more twins is further corroborated by the comparison of microstructure after rapture under both tensile (Fig.5) and compression conditions (Fig.4). Clearly, only a few twins are produced after compression, while comparatively large number of twins are presented after tension. Hence, under such circumstance, chances of work hardening is very large and the interaction between dislocations and twins are very necessary. It is natural that the ultimate stress under compression is about three times that of tension. What is more, with more twinning modes being possible, large plasticity is expected because inhomo- geneous deformation is accommodated.
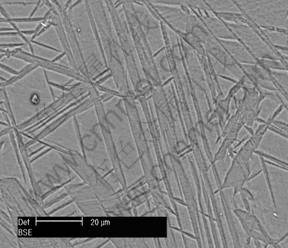
Fig. 4 Microstructure of LA40 after compression to rapture
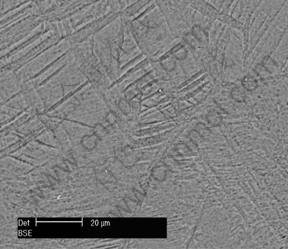
Fig.5 Microstructure of LA40 after tension to rapture
The actual causes for the onset of PLC effect in LA40 induced by tension are discussed elsewhere [21-22]. Summarily, it can be ascribed to dynamic strain aging (DSA) effect of Li atoms to mobile dislocations. However, when large quantities of twins are present, they may impose some influence on the DSA process. It might be the case that diffusion of Li atoms to mobile dislocations is greatly hampered by the presence of twins. As a consequence, there is no need for dislocation to be trapped by solute atoms and break through their surroundings. In sum, dynamic strain aging, which is the precursor of PLC effect, is impossible and serrations are smoothed out during compression.
5 Conclusions
The mechanical properties of LA40 magnesium- lithium alloy are studied under compressive deformation. Under such condition, this alloy exhibits high ultimate compressive strength and comparatively good plasticity. The PLC effect, predominant feature under tension, disappears. The observed phenomena are explained by the introduction of compression twins.
References
[1] DROZD Z, TROJANOVA Z, KUDELA S. Deformation behaviour of Mg-Li-Al alloys[J]. J Alloy and Compd, 2004, 378: 192-195.
[2] TROJANOVA Z, DROZD Z, LUKAC P, CHMELIK F. Deformation behaviour of Mg-Li alloys at elevated temperatures[J]. Mater Sci Eng A, 2005, 410/411: 148-151.
[3] YOSHIDA Y, CISAR L, KAMADO S, KOJIMA Y. Low temperature superplasticity of ECAE processed Mg-10%Li-1%Zn alloy[J]. Mater Trans, 2002, 43: 2419-2423.
[4] AGNEW S R, YOO M H, TOME N. Application of texture simulation to understanding mechanical behavior of Mg and solid solution alloys containing Li or Y[J]. Acta Mater, 2001, 49: 4277-4289.
[5] ALAMO A, BANCHIK D. Precipitation phenomena in the Mg-31 at.% Li 1 at.% alloy[J]. J Mater Sci, 1980, 15: 222-229.
[6] YIN D L, ZHANG K F, WANG G F, HAN W B. Warm deformation behavior of hot-rolled AZ31 Mg alloy[J]. Mater Sci Eng A, 2005, 392: 320-325.
[7] JAYAMATHY M, KAILAS S V, KUMAR K, SESHAN S, SRIVATSAN T S. The compressive deformation and impact response of a magnesium alloy: Influence of reinforcement[J]. Mater Sci Eng A, 2005, 393: 27-35.
[8] WATANABE H, TAKARA A, SOMEKAWA H, MUKAI T, HIGASHI K. Effect of texture on tensile properties at elevated temperatures in an AZ31 magnesium alloy[J]. Scripta Mater, 2005, 52: 449-454.
[9] GEHRMANN T, GRONMMERT M, GOTTSTEIN G. Texture effects on plastic deformation of magnesium[J]. Mater Sci Eng A, 2005, A395: 338-349.
[10] ISHIKAWA K, WATANABE H, MUKAI T. High strain rate deformation behavior of an AZ91 magnesium alloy at elevated temperatures[J]. Mater Lett, 2005, 59: 1511-1515.
[11] TROJANOVA Z, LUKAC P. Deformation behaviour of an AS21 alloy reinforced by short Saffil fibres and SiC particles[J]. J Mater .Proc Tech, 2005, 162/163: 416-421.
[12] AGNEW S R, MEHROTRA P, LILLO T M, STOICA G M, LIAW P K. Texture evolution of five wrought magnesium alloys during route A equal channel angular extrusion: Experiments and simulations[J]. Acta Mater, 2005, 53: 3135-3146.
[13] KESHAVARZ Z, BARNETT M R. EBSD analysis of deformation modes in Mg-3Al-1Zn[J]. Scripta Mater, 2006, 55: 915-918.
[14] JU D Y, HU X D. Effect of casting parameters and deformation on microstructure evolution of twin-roll casting magnesium alloy AZ31[J]. Trans Nonferrous Met Soc China, 2006, 16: 874-877.
[15] PRASAD Y V R K, RAO K P. Effect of crystallographic texture on the kinetics of hot deformation of rolled Mg-3Al-1Zn alloy plate[J]. Mater Sci Eng A, 2006, 432: 170-177.
[16] SANSCHAGRIN A, TREMBLAY R, ANGERS R, DUBE D. Mechanical properties and microstructure of new magnesium- lithium base alloys[J]. Mater Sci Eng A, 1996, 220: 69-77.
[17] HAFERKAMP H, JASCHIK C, JUCHMANN P, KAESE V, NIEMEYR M, TAI P. Development and properties of magnesium- lithium-alloys[J]. Materialwiss Werkstofftech, 2001, 32: 25-30.
[18] FROST P D, KURA J G, EASTWOOD L W. Aging characteristics of magnesium-lithium base alloys[J]. Trans AIME, 1950, 188: 1277-1282.
[19] YOO M H. Slip, twinning and fracture in hexagonal close-packed metals[J]. Metall Trans A, 1981, 12: 409-418.
[20] YOO M H, AGNEW S R, HO K M. Non-basal slip systems in HCP metals and alloys: source mechanisms[J]. Mater Sci Eng A, 2001, 319/321: 87-92.
[21] WANG C, XU Y B, HAN E H. Serrated flow and abnormal strain rate sensitivity of a magnesium-lithium alloy[J]. Mater Lett, 2006, 60: 2941-2944.
[22] WANG C, LI Z Q, XU Y B, HAN E H. Acoustic emission inspection of Portevin-Le Chatelier effect and deformation mechanisms of two Mg-Li-Al alloys[J]. J Mater Sci, (accepted).
(Edited by YUAN Sai-qian)
Foundation item: Project (50371089) supported by the National Natural Science Foundation of China
Corresponding author: HAN En-hou; Tel: +86-24-23971525; E-mail: ehhan@imr.ac.cn