J. Cent. South Univ. (2016) 23: 677-684
DOI: 10.1007/s11771-016-3113-1

Using structure restoration maps to comprehensively identify potential faults and fractures in compressional structures
Ou Cheng-hua(欧成华)1, Chen Wei(陈伟)1, Li Chao-chun(李朝纯)2
1. State Key Laboratory of Oil and Gas Reservoir Geology and Exploitation
(Southwest Petroleum University), Chengdu 610500, China;
2. State-owned Asset Management Office (Southwest Petroleum University), Chengdu 610500, China
Central South University Press and Springer-Verlag Berlin Heidelberg 2016
Abstract: Faults and fractures of multiple scales are frequently induced and generated in compressional structural system. Comprehensive identification of these potential faults and fractures that cannot be distinguished directly from seismic profile of the complex structures is still an unanswered problem. based on the compressional structural geometry and kinematics theories as well as the structural interpretation from seismic data, a set of techniques is established for the identification of potential faults and fractures in compressional structures. Firstly, three-dimensional (3D) patterns and characteristics of the faults directly interpreted from seismic profile were illustrated by 3D structural model. Then, the unfolding index maps, the principal structural curvature maps, and tectonic stress field maps were obtained from structural restoration. Moreover, potential faults and fractures in compressional structures were quantitatively identified relying on comprehensive analysis of these three maps. Successful identification of the potential faults and fractures in Mishrif limestone formation and in Asmari dolomite formation of Buzurgan anticline in Iraq demonstrates the applicability and reliability of these techniques.
Key words: potential fault and fracture; comprehensive identification; structure restoration maps; 3d structural modeling; compressional structures
1 Introduction
Much attention has been paid to nappe- compressional structures due to its high probability of storing oil and gas reserves. Many structural styles, including structural wedges [1], conjugate shear faults [2-3], fault growth related folds [1, 4], fold accommodation faults [5], oblique thrust fault [6-7], have been proposed to illustrate these systems. Generally, these structural styles engender various compressional faults and fractures, which could affect the development and production of the oil and gas reservoirs. Fault features are easily discernible on seismic profile, if they are of large scale. Meanwhile, these large faults and fractures frequently induce potential faults of small scale, which don’t display explicit features on the seismic profile, thus they are hard to be identified [8]. However, these small potential faults and fractures are crucial for oil and gas flow through the formation [9].
Therefore, identification of potential faults in the compressional structures is essential. Currently, most of the studies focus on the elaboration of the structural style and geometry, and kinematics and dynamics of the fault-related folds, the features of which are mostly apparent on the seismic profile. But studies on potential faults without distinct attributes are very scarce. In the present work, based on the 3D seismic data of high signal to noise ratio, a set of techniques is established to identify the potential faults and fractures in compressional structures, using 3D structural modeling and restoration. With these techniques, comprehensive identification of potential faults in Buzurgan anticline in the foreland basin of Zagros Mountain in Iraq has been successfully carried out.
2 Identification procedure
The flowchart of the comprehensive identification of potential faults in compressional structures restored from inversion of structure geometry deformation is shown in Fig. 1. The key techniques in this procedure are detailed as follows.
2.1 3D structural modeling
3D structural modeling is the prerequisite for the identification of the potential faults and fractures in a compressional structural system. First, the initial parameter field for fault groups and the formations that they pass through were generated upon the seismic data of high signal to noise ratio; then with surface modeling, the deformation distribution characteristics of the fault surface and the stratigraphic surface in 3D space were determined; eventually, utilizing the dissection features of the faults to the formations interpreted from fault-related fold theories [1, 3-5], spatial topological relations between the fault surface and the stratigraphic surface at various locations were built. Till this point, the 3D geometrical deformation characteristics, and the contact relations between the strata and faults have been precisely reconstructed.
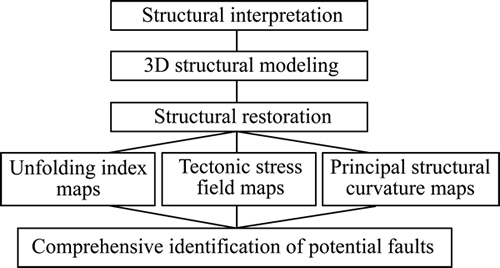
Fig. 1 Procedure of comprehensive identification of potential faults and fractures in complex compressional structures
Compressional structures in sedimentary basin usually present as conjugate shear faults [2-3], growth faults [1, 10], fold accommodation faults [5, 11], and etc. 3D structural modeling [12] primarily aims at precise reconstruction of the generation and inheritance of these compressional structures to further provide structural prototype for stratigraphic surface restoration.
2.2 Horizon surface restoration and map extraction
Unfolding and unfaulting processes were adopted to treat the deformed horizon surfaces and fault surfaces to eliminate the resultant structural deformation from the tectonic activities. Then, the deformation characteristics of the sedimentary formation at the early stage of paleotectonic activity were recovered, and the stress generation and distribution during the paleotectonic activities were revealed. White [13] first put forward the theory of simple shear, which was then used to restore horizon surfaces of tensional structures [14-15]. Due to the intrinsic difference between tensional and compressional structures, this method cannot be directly employed to restore the horizon surfaces in compressional structures. To resolve this mismatch, the modified inversion method of horizon line length [16], based on simple shear, was proposed for compressional structures by integrating structural analysis and balanced cross section techniques. This modified inversion method has been successfully applied to modeling and analyzing the structural geometry of the potential folding of late Indo Chinese epoch in the foreland of southern Longmen Mountain [17], and the geometric kinematics of the Dushanzi anticline in Xinjiang province [18].
This modified inversion method restored the structural horizons of the compressional structures, during which the unfolding index map, tectonic stress map and principal structural curvature map were extracted, accomplishing the prediction of development features and patterns for potential faults.
1) Unfolding index map.
Unfolding index (Iu) is the ratio of the compressional folding surface area before restoration Af to that after restoration Ah, as defined in eq. (1). It reflects the percentage of the folding that is eliminated. For rigid surface without internal deformation, Iu=1, taking a paper surface for example, the surface area before and after folding does not change. For structural horizon surfaces under compressional deformation, the surface area usually undergoes contraction, the magnitude of which represents the intensity of the compressional deformation. In other words, the surface contraction represents the probability of compressional faulting. Then, Iu was mapped to reflect the probability of faulting in compressional structures. The smaller the UI is, the more probable the faulting occurs.
(1)
2) Tectonic stress map.
Unfolding and unfaulting for stratigraphic horizon restoration essentially are processes releasing the paleotectonic stress exerted on the current structure, during which the stress vectors of various structures can be extracted. The maximum principal stress map indicates the magnitude and direction of maximum principal stress at different locations in the formation, displaying the superposed stress field of different paleostress sources on the compressional structure. In cases that lithological variation is small, namely, the rock mechanical behaviors are similar, according to the tectonic stress map, the location and orientation of the faults occurring perpendicular to the maximum horizontal stress can be easily determined.
3) Principal structural curvature map.
The maximum and minimum principal curvatures of a certain point were directly calculated from the horizon surface of 3D structural modeling, the larger absolute value of which was taken as the principal structural curvature. Principal structural curvature was mapped by sampling points over the horizon surface. In general, the area with large principal structural curvature tends to initiate faults, which has been widely used to predict the location of faulting development.
2.3 Comprehensive identification of potential faults
Due to the synergistic effect of the paleoclimate, paleodepth, geopressure differential, stress orientation, stress magnitude, lithological change, and so on, the compressional structure consisting of deformed horizons and faults is a highly nonlinear and severely heterogeneous system. Thus, each of the UI, tectonic stress, and principal structural curvature maps by itself is not able to cover all of these effects, and relatively accurate comprehensive identification can only be achieved by integrating the advantages of these three maps together.
Meanwhile, the above analyses also show that intrinsic relevance exists among the UI, tectonic stress, and principal structural curvature maps, in which the area with low UI is also the location where the tectonic stress concentrates, additionally it is the place of high principal structural curvature. Integration of these three maps is more favorable for the determination of the location and orientation of faults in the compressional structures, in comparison with each of them separately.
3 Case study
3.1 Region of interest
Buzurgan anticline is located in Missan province in Southeast Iraq, which is close to Iran. The anticline, with the axis in northwest to southeast parallel to Zagros Mountain, situates in the foreland basin of Zagros Mountain formed during the Himalayan orogeny. The anticline resulted from the horizontal compression of the Arabian plate moving northeast into the Eurasian plate, and thus is a compressional structural system [19-20]. Abundant of oil and gas reserves have been found in the Mishrif formation of the middle Cretaceous and the Asmari formation of the Oligocene [21-22].
The Mishrif formation that is primarily porous limestone is about 160 m thick. On the top, stable mudstone developed, forming well marked contrast. The Jaddala formation of Eocene above it, about 350 m thick, is comprised of interbedding ductile muddy limestone and chalk. It is the main decollement zone greatly affecting the shallow structure. Asmari formation, with the thickness of about 100 m, is mainly dolomite with locally distributed sandstone. The stable gypsum on the top is a marker bed for identifying the Asmari formation. The Fars formation developed in Miocene, about 500 m thick, is mainly constituted of gypsum and evaporates, serving as the local cap rock of the Buzurgan anticline.
3D seismic data were acquired over the entire Buzurgan anticline in 2013, with the element of 25 m× 25 m, sampling rate of 2 ms, basic frequency of 28 Hz, and dominant frequency in the range of 10-50 Hz, as shown in Figs. 2(a) and (b). The results processed from the seismic acquisition present relatively high signal to noise ratio, laying a solid foundation for the structural analysis of the Mishrif and Asmari formations in the Buzurgan anticline.
3.2 3D structural modeling and horizon surface restoration
Based on the seismic data of high signal to noise ratio, top boundaries of Mishrif, Jaddala, Asmari, and Lower Fars formations have been tracked; meanwhile, ten faults are interpreted according to the seismic event identification, as shown in Figs. 2(a) and (c). As can be seen from the seismic section, two types of structural deformation developed above and below the Jaddala decollement zone. Formations below, affected by the early Cenozoic Zagros orogeny, yield nearly symmetric broad anticline with good continuity for the seismic events and relative consistent thickness.
As the Zagros orogeny intensified during the late Himalayan movement, immense compressional stress transited along the Jaddala decollement zone to the southwest. This extrusion folded the internal layers in Jaddala formation and created piercements in certain regions, breaking the continuity of the overlying formations and causing large variations in the thickness. During the horizontal slip, the overlying formation underwent varying ruptural deformations, modifying the local structure by thrusting up in the flank and anticlinal parts. Series of fold accommodation faults were generated with the orientation roughly consistent with the northwest and southeast Zagros Mountain, slightly tending to the southwest [22-23]. Since the Buzurgan anticline situates in the foreland basin of Zagros, the structural deformation is relatively weak, and the scale of the fold accommodation faults is small. Except the faults interpreted from the explicit imaging discontinuity, in other areas with only distortion, it is difficult to directly identify the existing potential faults.
Geometrical data of horizons and faults interpreted from seismic section are loaded into 3D structural modeling software to build the 3D model for Buzurgan anticline (Figs. 3 and 4), which reproduces the spatial pattern and intersection of the horizons and faults. Ten faults interpreted are mainly fold accommodation faults and lie between the top of Asmari and the top of Lower Fars, and there is no faults below the top surface of Jaddala. Most of these faults (F1-F3, F5, F6, and the northwest part of F7) situate in the southwest wing of the anticline, part of them (F8-F10) lie in the northwest end, F4 and the southeast part of F7 is located near the center of the anticline, as shown in Figs. 2(a), Figs. 3 and 4.
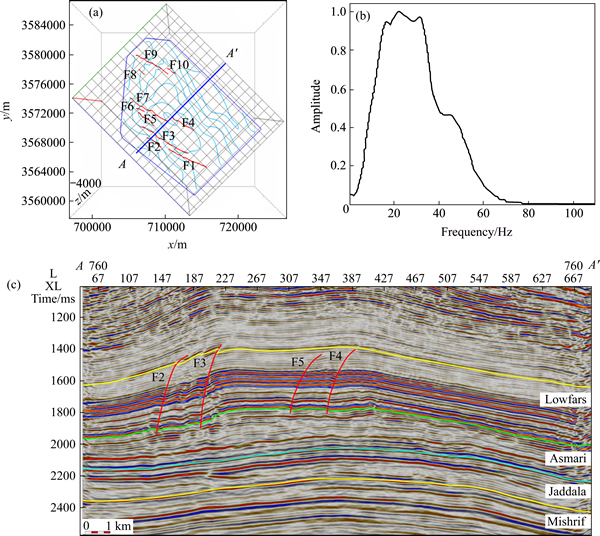
Fig. 2 Location of Buzurgan anticline and faults distribution (a), amplitude frequency response (b), seismic section and interpretation (c)
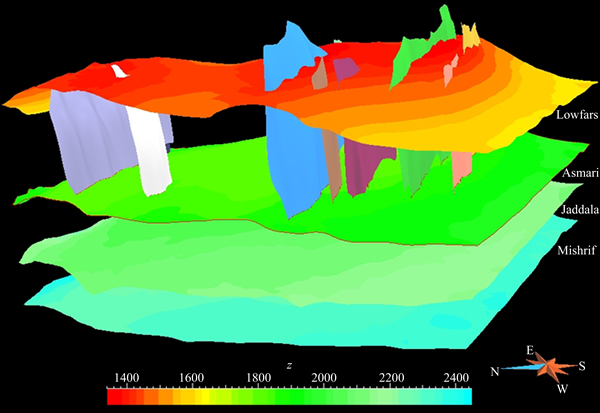
Fig. 3 3D structural model of Buzurgan anticline
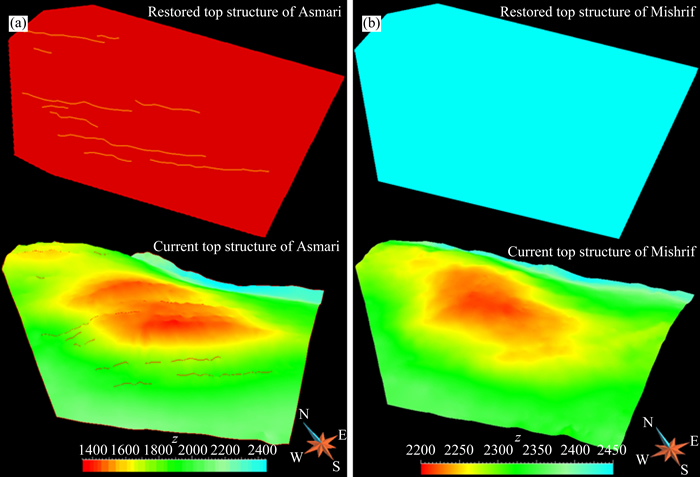
Fig. 4 Current (lower) and restored (up) top structures of Asmari (a) and Mishrif (b) formations in Buzurgan anticline
The top surfaces of Asmari and Mishrif formations in Buzurgan anticline were restored on the basis of 3D structural model and the abovementioned method, and results are compared with current structure, as shown in Fig. 4. It is obvious that the restored surfaces remain generally horizontal, and the faults in the upper and lower sides match well. These interpretations conform to the geological features of the compressional structures, and in turn demonstrate the applicability of horizon restoration method to compressional structural system.
3.3 Analysis and discussion of potential faults
Unfolding index, principal structural curvature and tectonic stress maps for the top surfaces of Asmari and Mishrif in Buzurgan anticline were extracted through structural restoration, providing the parameter set for potential fault analysis and discussion, as shown in Fig. 5.
1) Map appearance of interpreted faults.
Comparison of the locations of faults F1-F10 in Figs. 1-4 to the UI, principal structural curvature and tectonic stress maps in Fig. 5 shows that: interpreted faults F1-F10 correspond one-to-one to the low values indicated by the red-green-light blue colors in the UI map, while cyan-purple-white colors representing high values indicate undeveloped faults; faults F1-F10 also agree very well with the purple-dark blue-light blue colors of high values in the principal structural curvature map, whereas white-yellow-red colors of low values reflect undeveloped faults; from the tectonic map superposing the maximum stress and the stress field, correspondence exists between the maximum stress concentrated area and the interpreted faults. Besides, the stress trajectory manifests the primary northeast- southwest compression, which is consistent with the tectonic setting of this area. One-to-one correspondence relations can also be found in terms of careful examination of the faults of inline 760 seismic section in Fig. 2 with these three maps.
These results fully confirm the consistency among the three maps of UI, principal structural curvature and tectonic stress, and the interpreted faults, which makes up the basis for potential faults analysis by integrating these three maps.
2) Prediction of potential faults and fractures.
According to the above analyses, the area overlapped by the red-green-light blue colors of low UI and the purple-dark blue-light blue colors of high principal curvature is where the potential faults developed, and the direction perpendicular to the local maximum horizontal stress indicates the orientation of the potential faults extending.
Thereby, it is predicted that the potential faults in Asmari formation of Buzurgan anticline mainly distribute in the southwest wing, the southwest part of the anticline center, and the northwest end (Figs. 5(a), (c) and (e)), which are also the dense distribution areas of interpreted faults (Figs. 3 and 4). These potential faults were primarily induced and are in alignment with the fold accommodation faults as interpreted above. The potential faults in Mishrif formation merely scattered in the southwest wing and parts of the northeast wing of the anticline (Figs. 5(b), (d) and (f)).
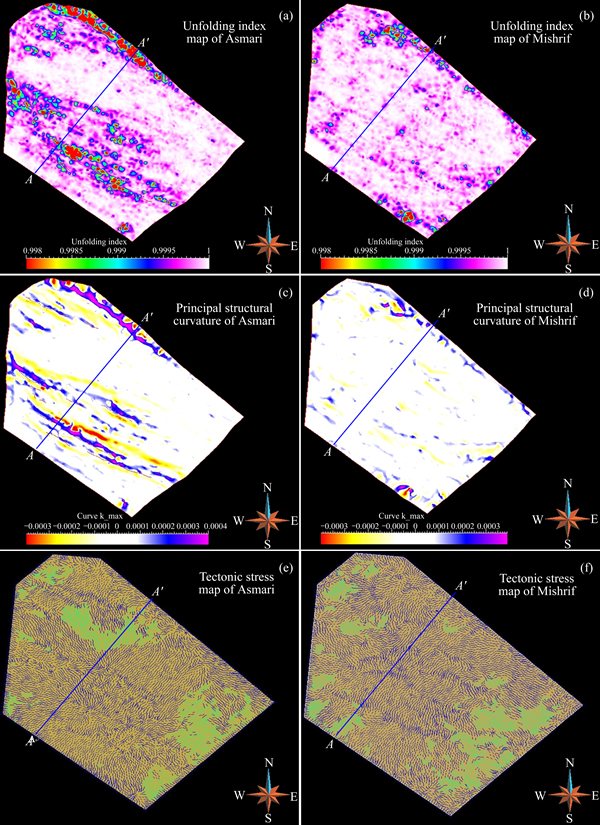
Fig. 5 Unfolding index maps (a, b), principal structural curvature (c, d) and tectonic stress maps (e, f) for Asmari (a, c, e) and Mishrif (b, d, f) formations in Buzurgan anticline
Relying on structural interpretation and comprehensive identification of potential faults from UI, principal structural curvature and tectonic stress maps in Fig. 5, top structures of Asmari and Mishrif formations were rebuilt, as shown in Figs. 6(a) and (c). In Fig. 6(a), the faults interpreted above were changed properly according to the results of identification of potential faults; and in Fig. 6(c), no faults were found as mentioned before. To confirm the reliability of the above mentioned results, coherent time slices close to top surfaces of Asmari and Mishrif (Figs. 6(b) and (d)) were picked up. By comparing the fault features in these three maps (Fig. 5), top structure maps (Figs. 6(a) and (c) and coherent time slices (Figs. 6(b) and (d)) were obtained. The same potential faults information can be found from the coherent time slices, which is the best proof of the validity of the identification procedure.
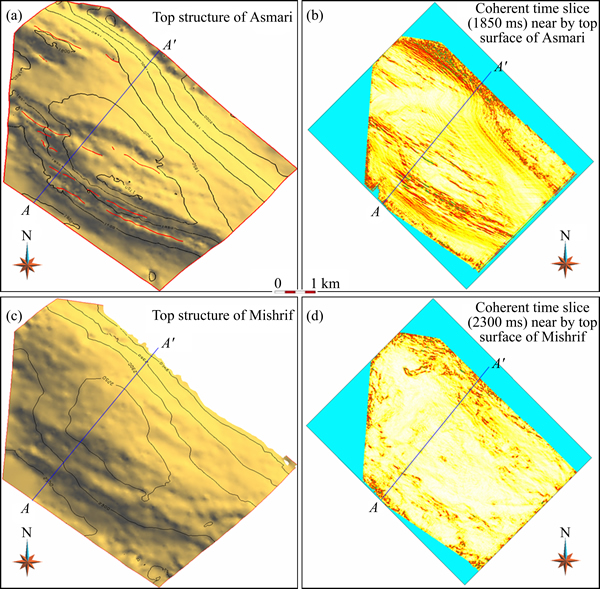
Fig. 6 Top structures of Asmari (a) and Mishrif (c) obtained from structural interpretation and comprehensive identification of potential faults, coherent time slice close to top surfaces of Asmari (b) and Mishrif (d)
3) Marginal singularity of seismic section.
It should be noted that because of the low credibility of the marginal seismic data, especially the irregular disturbance and chaotic reflection shown in Fig. 2(c), horizon restoration suffers from certain deviations, which bring up false faults at these singular locations in the UI and principal structural curvature maps. For instance, evident singularity appears from top to bottom along the northeast margin of inline 760 seismic section in Fig. 2(c), generating large amount of false faults in the northeast margin in the UI in Figs. 5(a) and (b) and principal structural curvature maps in Figs. 5(c) and (d). Here it can be seen that the false faults can be easily identified form the seismic section, and they do not affect the effectiveness and reliability of the method proposed here for potential faults identification in compressional structural systems.
4 Conclusions
1) Based on inversion and restoration of geometrical deformation, a set of comprehensive identification techniques for potential faults in compressional structural systems have been set up. According to the 3D compressional structural model, this methodology first extracts the UI, principal structural curvature and tectonic stress maps through structural horizon restoration, and then integrates them together to analyze and predict the potential faults in the compressional structural systems.
2) This methodology successfully predicted the potential faults in the Buzurgan anticline structure in Iraq, which in turn demonstrates the feasibility and reliability of the method, opening up a new way for potential faults prediction in compressional structural systems.
Acknowledgement
The authors are grateful for the instructive discussions with Deng Yun-hua from the research institute of CNOOC and Wang Xing from CNOOC-Iraq Company. The authors also appreciate the help from Gao Yun-feng, Luo Yin-fu, Yang Yu-gui and Zhang Yu-kun at the research institute of CNOOC and Zhou Shou-xin for providing the basic data for this research.
References
[1] Shaw J H, Connors C D, Suppe J. Seismic interpretation of contractional fault-related folds: An AAPG seismic atlas [M]. American Association of Petroleum Geologists, 2005: 46-87.
[2] Ferrill D A, Morris A P, McGinnis R N. Crossing conjugate normal faults in field exposures and seismic data [J]. AAPG Bulletin, 2009, 93(11): 1471-1488
[3] Olsson W A, Lorenz J C, Cooper S P. A mechanical model for multiply-oriented conjugate deformation bands [J]. Journal of Structural Geology, 2004, 26(2): 325-338.
[4]
J, Poblet J, Roca E,
J. Bed-by-bed fold growth by kink-band migration: Sant
de Morunys, eastern Pyrenees [J]. Journal of Structural Geology, 1997, 19(3): 443-461.
[5] Wrede V. Thrusting in a folded regime: fold accommodation faults in the Ruhr basin, Germany [J]. Journal of structural geology, 2005, 27(5): 789-803.
[6] Sylvester A G. Strike-slip faults [J]. Geological Society of America Bulletin, 1988, 100(11): 1666-1703.
[7] Santoro E, Ferranti L, Burrato P, MAZZELLA M E, MONACO C. Deformed Pleistocene marine terraces along the Ionian Sea margin of southern Italy: Unveiling blind fault-related folds contribution to coastal uplift [J]. Tectonics, 2013, 32(3): 737-762.
[8] OU Cheng-hua, CHEN Wei, MA Zhong-gao. Quantitative identification and analysis of sub-seismic extensional structure system: technique schemes and processes [J]. Journal of Geophysics and Engineering, 2015, 12(3): 502-514.
[9] OU Cheng-hua. Fluid typing extends production in Chinese gas reservoir [J]. Oil & Gas Journal, 2015, 113(2): 54-61.
[10] WHITE N. A method for automatically determining normal fault geometry at depth [J]. Journal of Geophysical Research: Solid Earth, 1992, 97(B2): 1715-1733.
[11] Giambiagi L, Ghiglione M, Cristallini E, BOTTESI G. Kinematic models of basement/cover interaction: Insights from the Malargüe fold and thrust belt, Mendoza, Argentina [J]. Journal of Structural Geology, 2009, 31(12): 1443-1457.
[12] OU Cheng-hua, WANG Xiao-lu, LI Chao-chun, HE Yan. Three-dimensional modelling of a multi-layer sandstone reservoir: The Sebei Gas Field, China [J]. Acta Geologica Sinica: English Edition, 2016, 90(1): 801-840.
[13] White N. A method for automatically determining normal fault geometry at depth [J]. Journal of Geophysical Research: Solid Earth (1978-2012), 1992, 97(B2): 1715-1733.
[14] Durand-Riard P, Caumon G, Muron P. Balanced restoration of geological volumes with relaxed meshing constraints [J]. Computers & Geosciences, 2010, 36(4): 441-452.
[15] Song T, Cawood P A. Effects of subsidiary faults on the geometric construction of listric normal fault systems (Geologic Note) [J]. AAPG bulletin, 2001, 85(2): 221-232.
[16] CHEN Wei, YANG Yang. Computer simulation of fault geometric shape inversion [J]. Computing Techniques for Geophysical and Geochemical Exploration, 2008, 30(6): 490-493. (in Chinese)
[17] CHEN Wei, YANG Ke-ming, LI Shu-bin, JIAN Gao-ming, TANG Jing, ZHAO Yao, SU Qiang. The geometric simulation and analysis of the blind growth fold in the frontier overthrust belt of the southern section of the Longmenshan during Late Triassic [J]. Chinese Science Bulletin, 2009, 54(8): 382-386. (in Chinese)
[18] CHEN Wei, HAO Jin-jin, LI Shi-qin, PENG Wen-li, XIAN Di, CHEN Li-hua, LI Zhi-gang. The geometric and kinematic numerical simulation of the Dushanzi anticline, southern Junggar Basin [J]. Chinese Journal of Geology, 2012, 47(1): 37-50. (in Chinese)
[19] Stoneley R. The Arabian continental margin in Iran during the Late Cretaceous [J]. Geological Society, London, Special Publications, 1990, 49(1): 787-795.
[20] Pirajno F. China’s tectonic framework in the global context [M]// The Geology and Tectonic Settings of China’s Mineral Deposits. Netherlands: Springer, 2013: 19-33.
[21] Ameen M S. Effect of basement tectonics on hydrocarbon generation, migration, and accumulation in Northern Iraq (1) [J]. AAPG Bulletin, 1992, 76(3): 356-370.
[22] HAN Yao-zu, CHEN Wei, OU Cheng-hua, ZHOU Xiao-jun, LI Shi-qin. Geometric analysis and kinematic simulation of Abu Ghirab anticline in Missan area, Iraq [J]. Xinjiang Petroleum Geology, 2014, 35(1): 124-129. (in Chinese)
[23] GUAN Shu-wei, LI Ben-liang, HE Deng-fa, WANG Xin. Geometrical methods of complicated structural analysis and their application [J]. Chinese Journal of Geology, 2007, 42(4): 722-739. (in Chinese)
(Edited by YANG Hua)
Foundation item: Project(2014CB239205) supported by the National Basic Research Program of China; Project(20011ZX05030-005-003) supported by the National Science and Technology Major Project of China
Received date: 2014-12-24; Accepted date: 2015-04-03
Corresponding author: Ou Cheng-hua, Professor, PhD; Tel: +86-13551030708; E-mail: chomm@163.com