J. Cent. South Univ. (2014) 21: 1714-1718
DOI: 10.1007/s11771-014-2114-1

TiO2/graphene nanocomposites as anode materials for high rate lithium-ion batteries
TANG Yi-ping(唐谊平), WANG Shi-ming(王诗明), TAN Xiao-xu(谭晓旭),HOU Guang-ya(侯广亚), ZHENG Guo-qu(郑国渠)
College of Chemical Engineering and Material Science, Zhejiang University of Technology, Hangzhou 310014, China
Central South University Press and Springer-Verlag Berlin Heidelberg 2014
Abstract: A simple strategy to prepare a hybrid of nanocomposites of anatase TiO2/graphene nanosheets (GNS) as anode materials for lithium-ion batteries was reported. The morphology and crystal structure were studied by X-ray diffraction (XRD), field emission scanning electron microscopy (FE-SEM) and transmission electron microscopy (TEM). The electrochemical performance was evaluated by galvanostatic charge–discharge tests and alternating current (AC) impedance spectroscopy. The results show that the TiO2/GNS electrode exhibit higher electrochemical performance than that of TiO2 electrode regardless of the rate. Even at 500 mA/g, the capacity of TiO2/GNS is 120.3 mAh/g, which is higher than that of TiO2 61.6 mAh/g. The high performance is attributed to the addition of graphene to improve electrical conductivity and reduce polarization.
Key words: TiO2; graphene nanosheets; lithium-ion batteries; anode materials
1 Introduction
Titanium oxide (TiO2) is a widely studied semiconductor metal oxide due to its great application potential in many fields, such as pigments, photocatalysis, sensors and solar cells [1-4]. TiO2 has been intensively studied as the anode material for lithium-ion batteries because of its low cost, abundance, non-toxicity and structural stability during lithium insertion/extraction [5-6]. TiO2 has various polymorphs, such as rutile, anatase, brookite and TiO2(B). Among these anatase and TiO2(B) are more electrochemical active than others [7]. The performance of TiO2 has great relation with its crystalline phase, surface state and microstructure. The varied morphologies of TiO2 include nanotubes, nanowires, naonorods and nano crystallines [8]. TiO2 composites with other materials can also improve its performance. For example, TiO2/MnO2 nanohybrid was obtained through a pillaring method [9], this hybrid shows an enhanced specific capacity of 288 mAh/g, which is much higher than the theoretical value of 193 mAh/g. This is attributed to the large specific surface produced by the pillaring reaction. The TiO2/MoO3 was prepared to enhance lithium storage capacity and cyclic performance by PAEK et al [10]. They did this job via an exfoliation and reassembling method. The TiO2/ MoO3 had a new porous heterostructure. The reversible capacity of the hybrid was as high as 420 mAh/g. These examples indicate that TiO2 was widely used in lithium batteries in varied forms. However, the low Li-ion diffusivity and electronic conductivity severely deteriorate its reversible capacity and high rate performance [11], which limits its application and development.
Due to the excellent properties such as an extraordinary electronic transport property, a flexible structure, high mechanical strength and high surface area, graphene nanosheets (GNS) is regarded as an ideal conductive additive to nanostructured hybrids as electrodes of LIBs [12-18]. Many metal oxides/GNS composite electrodes were reported such as Mn3O4/GNS, Fe3O4/GNS and Co3O4/GNS, the results showed that the addition of GNS can not only increase the electrical conductivity, but also improve the capacity and cyclic stability of anode materials [11-13]. Recently, the nanocomposite of Li4Ti5O12/GNS was prepared as anode materials for LIBs, which showed great improved surface conductivity as well as rate capability [19-20]. It can be inferred that the nanocomposite of TiO2/GNS has perfect electrochemical performance.
A hybrid of nano-TiO2 that was well dispersed on GNS as an anode material for LIBs was prepared. It is found that the nanocomposite exhibits significantly improved rate capability and cycling performance.
2 Experimental
2.1 Material preparation
Figure 1 show the schematic of the synthesis routes of TiO2/GNS nanocomposites. GNS were prepared from natural flake graphite powder by chemical exfoliation as reported elsewhere [21]. The natural flake graphite powder was used to prepare graphite oxide by Hummers method [22]. Then, thermally expanded graphene oxide (GO) was synthesized by fast thermal expansion/ exfoliation of the graphite oxide (1323 K, Ar, 30 s). Finally, hydrogen reduction of GO (673 K, 2 h) to obtain GNS. A uniformly dispersed GNS solution using N-methyl-2- pyrrolidine (NMP) as dispersant was mixed with the anatase TiO2 nanoparticles (10-20 nm, Aladdin Reagent Co., Ltd.) to give 5% (mass fraction) GNS in the hybrid, which was used for most of the study unless otherwise mentioned. After 1 h vigorous stirring, the mixture was heated at 473 K to remove the solvent and ground in a mortar. Finally, a grey powder of TiO2/GNS hybrid was obtained.
2.2 Material characterization
X-ray diffraction was performed with a Philips Panalytical X’pert diffractometer using Cu Kα radiation. The morphology was observed by a HITACHI S-4700 scanning electron microscope (SEM) and a JEOL 2010 transmission electron microscope (TEM). All electrochemical measurements were tested by using a CR2032 coin-type two-electrode cells assembled in a glove box under argon atmosphere. The working electrode was fabricated by mixing the active material (TiO2/GNS), acetylene black and polyvinylidene fluoride (PVDF) binder in the mass ratio of 8:1:1. The lithium foil was used as both counter electrode and reference electrode. A 1 mol/L solution of LiClO4 in ethylene carbonate (EC)-dimethyl carbonate (DME) (1:1 in volume) was used as the electrolyte with a polypropylene membrane (Cellgard 2400) as the separator. The samples were heated to 393 K in a vacuum and kept 12 h to remove adsorbed water on the surface. Cell assembly was carried out in an Ar-filled glove box with the water and oxygen content less than 1×10-6.
Galvanostatic charge/discharge measurements were performed on a Land Battery Test System (CT2001A, Wuhan Land Electronic Co. Ltd., China) with an accuracy of 0.1% for controlling currents and measuring potentials in a potential range of 0-3.0 V. Electrochemical impedance spectroscopy (EIS) was conducted on a CHI660B electrochemical workstation with the frequency ranging from 0.01 Hz to 100 kHz. All the electrochemical tests were carried out at room temperature (298 K).
3 Results and discussion
3.1 Structure and morphology of materials
The X-ray diffraction (XRD) spectra of the samples are shown in Fig. 2. All peaks of TiO2 nanoparticles can be indexed as pure anatase according to JCPDS No. 21-1272 and no impurity peaks can be found. The as- prepared TiO2/GNS nanocomposite retains the same position of the diffraction peaks of TiO2 nanoparticles. It indicates that the addition of GNS has no effect on the crystal structures of TiO2 nanoparticles. The grain sizes of TiO2 and TiO2/GNS are estimated by Scherrer’s formula based on the (101) peak, which are 13.5 and 14.3 nm, respectively. It confirms that the special structure of nanosheet of GNS prevents the TiO2 particle from coalescence during the preparation process.
Figure 3(c) presents the TEM images of TiO2/GNS nanocomposites synthesized from GO. It is evident that two-dimensional GNS are well decorated by a large quantity of spherical TiO2 nanostructures, and both the outline of GNS and TiO2 nanoparticles can be clearly observed. The high-resolution TEM image (Fig. 3(d)) indicates that the crystal lattice fringes with d-spacing of 0.35 nm can be assigned to the (101) plane of the anatase,which is consistent with the XRD results. This provides a further evidence for the phase purity, while the GNS firmly contact with the TiO2 particles, giving a better connection between adjacent TiO2 particles, as well as preventing them from further aggregation.
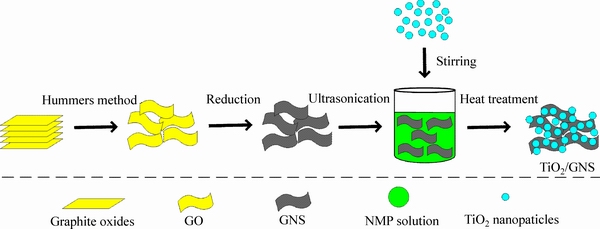
Fig. 1 Schematic of synthesis routes of TiO2/GNSs nanocomposites
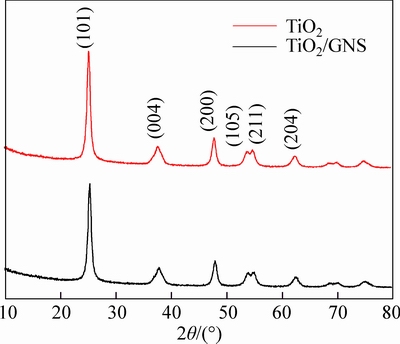
Fig. 2 XRD patterns of TiO2 and TiO2/GNS nanocomposite
3.2 Electrochemical performance
Figure 4 shows the charge and discharge curves of the TiO2 and TiO2/GNS nanocomposite electrodes at different rates from 50 to 500 mA/g. For the TiO2/GNS nanocomposite electrodes shown in Fig. 4(b), the initial discharge capacity is 787.9 mAh/g at 50 mA/g, which is much higher than 284.1 mAh/g for the TiO2 electrode as shown in Fig. 4(a). However, when the current density is 100 mA/g, the capacity of TiO2/GNS electrode drops sharply to 389 mAh/g. With the increase of current density from 100 to 500 mA/g, the capacity of all samples decreases. In addition, the TiO2/GNS nanocomposite always shows a higher discharge capacity than the TiO2 regardless of the rate. Even at 500 mA/g, the capacity of TiO2/GNS is 120.3 mAh/g, which is higher than that of TiO2 61.6 mAh/g. From these charge/discharge curves, it can be seen that when charged/discharged at low rates both electrodes exhibit a flat operation potential plateau. However, with the rate increased, the potential plateau of the TiO2 becomes shorter and gradually bends down while that of the TiO2/GNS electrode still remains flat.
Figure 5 shows the results of the rate capability test conducted at the different current densities of 50, 100, 200, 300, and 500 mA/g. As the current density increases, the capacity of all samples decreases. In particular, the TiO2/GNS electrodes exhibit higher rate performance than TiO2 electrodes at all current densities. At 50, 100, 200, 300, and 500 mA/g, the average capacities of TiO2/ GNS electrodes are 342.6, 214, 158, 136.8, 114 mAh/g, respectively, and the capacities of TiO2 electrodes are 210.9, 142, 98.9, 76.2, 59.2 mAh/g, respectively. The improvement of rate capability of the TiO2/GNS electrodes could be attributed to the effects of graphene by reducing resistance and polarization. With a graphene conductive network throughout the whole hybrid material, more pathways for electron transport are produced, thus the electron transport is more effective and the electrical conductivity of the electrode is improved.
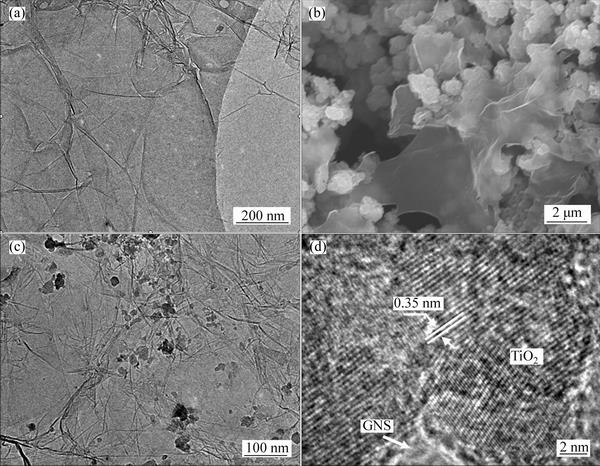
Fig. 3 TEM images of GNS (a), and SEM (b), TEM (c) and HRTEM (d) images of TiO2/GNS nanocomposite
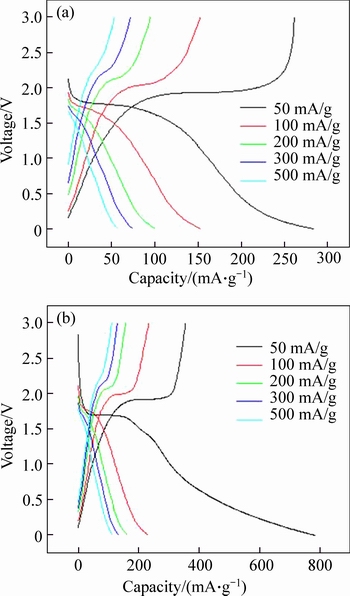
Fig. 4 Charge and discharge curves of TiO2 (a) and TiO2/GNS (b) electrodes at different current densities between voltage limits of 0-3 V
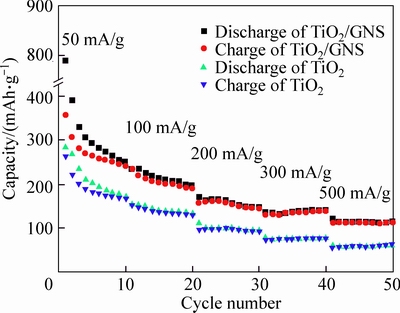
Fig. 5 Rate capability of TiO2 and TiO2/GNS electrodes at different current densities between voltage limits of 0-3 V
Figure 6 presents typical Nyquist plots obtained after the rate performance experiments for TiO2 and TiO2/GNS electrodes. The plots are similar to each other in shape, with a semicircle appearing in the high frequency domain and a straight line in the low frequency region. Some of the parameters obtained by fitting are listed in Table 1. It shows that both Rs and Rct of the TiO2/GNS electrode are smaller than those of TiO2 electrode, whereas the exchange current density (i0=RT/(nFRct)) of the TiO2/GNS electrode is higher than that of TiO2 electrode. Therefore, the charge transfer reaction of the TiO2/GNS electrode takes place rather than TiO2 electrode. Since the faradaic reaction is determined by ion transfer and electron conduction, the reduction of resistance might be attributed to the improved electronic conductivity of the addition of graphene, though it was only 5% in the nanocomposites.
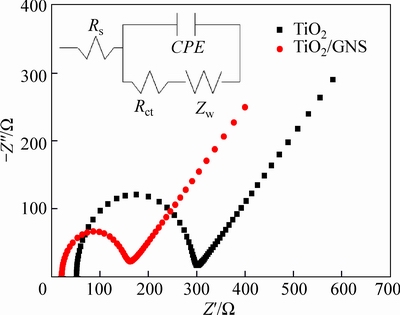
Fig. 6 AC impedance results for TiO2 and TiO2/GNS electrodes obtained after tests of rate performance (The equivalent circuit for the AC impedance spectra is depicted in inset. Rs is electrode resistance, Rct is charge-transfer resistance, respectively, CPE is double layer capacitance, and ZW is Warburg impedance)
Table 1 Impedance parameters of TiO2/GNS and TiO2 anodes
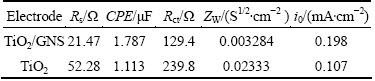
4 Conclusions
1) The nanocomposite of TiO2/GNS is prepared from natural flake graphite powder by chemical exfoliation as reported elsewhere.
2) The crumpled and rippled structure of GNS gives a better connection between adjacent TiO2 particles, preventing them from further aggregation, and helps to improve the electrical conductivity and reduce the polarization of the nanocomposites.
3) The TiO2/GNS electrode exhibits higher electrochemical performance than that of TiO2 electrode regardless of the rate. Even at 500 mA/g, the capacity of TiO2/GNS is 120.3 mAh/g, which is higher than that of TiO2 61.6 mAh/g.
4) Both Rs and Rct of the TiO2/GNS electrode are smaller than those of TiO2 electrode. Whereas the TiO2/GNS nanocomposite shows a higher exchange current density and discharge capacity than that of TiO2 regardless of the rate.
5) With a graphene conductive network throughout the whole hybrid material, more pathways for electron transport are produced, thus the electron transport is more effective and the electrical conductivity of the electrode is improved.
References
[1] CHEN X B, MAO S S. Titanium dioxide nanomaterials: Synthesis, properties, modifications and applications [J]. Chemical Reviews, 2007, 107(7): 2891-2959.
[2] DENG D, KIM M G, LEE J Y, CHO J. Green energy storage materials: Nanostructured TiO2 and Sn-based anodes for lithium-ion batteries [J]. Energy & Environmental Science, 2009, 2(8): 818-837.
[3] Subramanian V, Karki A, Gnanasekar K I, Eddy F P, RAMBABU B. Nanocrystalline TiO2 (anatase) for Li-ion batteries [J]. Journal of Power Sources, 2006, 159(1): 186-192.
[4] DAS S K, DARMAKOLLA S, Bhattacharyya A J. High lithium storage in micrometre sized mesoporous spherical self- assembly of anatase titania nanospheres and carbon [J]. Journal of Materials Chemistry, 2010, 20(8): 1600-1606.
[5] WANG X Y, XIE K Y, LI J, LAI Y Q, ZHANG Z A, LIU Y X. Synthesis and electrochemical performance of TiO2-B as anode material [J]. Journal of Central South University of Technology, 2011, 18(2): 406-410.
[6] CAHEN D, HODES G, Gratzel M, GUILLEMOLES J F, RIESS I. Nature of photovoltaic action in dye-sensitized solar cells [J]. The Journal of Physical Chemistry B, 2000, 104(9): 2053-2059.
[7] KAVAN L, ATTIA A, LENZMANN F, ELDER S H, GRATZEL M. Lithium insertion into zirconia-stabilized mesoscopic TiO2 (anatase) [J]. Journal of the Electrochemical Society, 2000, 147(8): 2897- 2902.
[8] Yang Zhen-guo, Choi D, Kerisit S, ROSSO K M, WANG Dong-hai, ZHANG J. Nanostructures and lithium electrochemical reactivity of lithium titanites and titanium oxides: A review [J]. Journal of Power Sources, 2009, 192(2): 588-598.
[9] KANG J H, PEAK S M, HWANG S J, CHOY J H. Pre-swelled nanostructured electrode for lithium ion battery: TiO2-pillared layered MnO2 [J]. Journal of Materials Chemistry, 2010, 20, 2033- 2038.
[10] PEAK S M, KANG J H, JUNG H, HWANG S J, CHOY J H. Enhanced lithium storage capacity and cyclic performance of nanostructured TiO2–MoO3 hybrid electrode [J]. Journal of Chemistry Communication, 2009, 7536-7538.
[11] Ren Y, Hardwick L J, Bruce P G. Lithium intercalation into mesoporous anatase with an ordered 3D pore structure [J]. Angewandte Chemie-International Edition, 2010, 122(14): 2624- 2628.
[12] Zhou Guang-min, WANG Da-wei, LI Feng, ZHANG Li-li, LI Na, WU Zhong-shuai, WEN L, LU Gao-qing, CHENG Hui-ming. Graphene-wrapped Fe3O4 anode material with improved reversible capacity and cyclic stability for lithium ion batteries [J]. Chemistry of Materials, 2010, 22(18): 5306-5313.
[13] WANG Hai-liang, CUI Li-feng, YANG Yuan, CASALONGUE H S, ROBINSON J T, LIANG Yong-ye, Cui Y, DAI Hong-jie. Mn3O4-graphene hybrid as a high-capacity anode material for lithium ion batteries [J]. Journal of the American Chemical Society, 2010, 132(40): 13978-13980
[14] WU Zhong-shuai, REN Wen-cai, WEN Lei, GAO Li-bo, ZHAO Jin-ping, CHEN Zong-ping, ZHOU Guang-min, LI Feng, CHENG Hui-Ming. Graphene anchored with Co3O4 nanoparticles as anode of lithium ion batteries with enhanced reversible capacity and cyclic performance [J]. ACS Nano, 2010, 4(6): 3187-3194.
[15] PAEK S M, YOO E, Honma I. Enhanced cyclic performance and lithium storage capacity of SnO2/graphene nanoporous electrodes with three-dimensionally delaminated flexible structure [J]. Nano Letters, 2009, 9(1): 72-75.
[16] WANG Dong-hai, CHOI D W, LI Juan, YANG Zhen-guo, NIE Zi-min, KOU Rong, HU De-hong, WANG Chong-min, Saraf L V, ZHANG Ji-guang, AKSAY I A, LIU Jun. Self-assembled TiO2-graphene hybrid nanostructures for enhanced Li-ion insertion [J]. ACS Nano, 2009, 3(4): 907-914.
[17] Liang Ming-hui, Zhi Lin-jie. Graphene-based electrode materials for rechargeable lithium batteries [J]. Journal of Materials Chemistry, 2009, 19(31): 5871-5878.
[18] Stankovich S, Dikin D A, Dommett G H B, KOHLHAAS K M, ZIMNEY E J, STACH E A, PINER R D, NGUYEN S T, RUOFF R S. Graphene-based composite materials [J]. Nature, 2006, 442: 282-286.
[19] ShI Ying, Wen Lei, Li Feng, CHENG Hui-Ming. Nanosized Li4Ti5O12/graphene hybrid materials with low polarization for high rate lithium ion batteries [J]. Journal of Power Sources, 2011, 196(20): 8610-8617.
[20] Zhu Nan, Liu Wen, XUE Mian-qi, XIE Zhuang, ZHAO Dan, ZHANG Mei-ning, CHEN Ji-tao, CAO Ting-bing. Graphene as a conductive additive to enhance the high-rate capabilities of electrospun Li4Ti5O12 for lithium-ion batteries [J]. Electrochimica Acta, 2010, 55(20): 5813-5818.
[21] WU Zhong-shuai, REN Wen-cai, GAO Li-bo, LIU Bi-lu, JIANG Chuan-bin, CHENG Hui-Ming. Synthesis of high-quality graphene with a pre-determined number of layers [J]. Carbon, 2009, 47(2): 493-499.
[22] BAI Hua, XU Yu-xi, ZHAO Lu, LI Chun, SHI Gao-quan. Non covalent functionalization of graphene sheets by sulfonated [J]. Chemical Communications, 2009, 13: 1667-1669.
(Edited by HE Yun-bin)
Foundation item: Project(Y4110230) supported by Natural Science Foundation of Zhejiang Province, China; Project(51204146, 51101140) supported by the National Natural Science Foundation of China; Project(2012M521197) supported by Postdoctoral Science Foundation of China
Received date: 2012-12-18; Accepted date: 2013-05-10
Corresponding author: ZHENG Guo-qu, Professor, PhD; Tel: +86-571-88320429; E-mail: zhenggq@zjut.edu.cn