
Effect of ultrasonic on structure and electrochemical performance of α-Ni(OH)2 electrodes
ZHANG Zhong-ju, ZHU Yan-juan, BAO Jie, ZHOU Zhuo-jun, YE Xian-cong, XU Qing-sheng
School of Physics and Optoelectronic Engineering, Guangdong University of Technology, Guangzhou 510006, China
Received 9 December 2010; accepted 16 May 2011
Abstract: Al/Co co-doped α-Ni(OH)2 samples were prepared by either ultrasonic co-precipitation method (Sample B) or co-precipitation method (Sample A). The crystal structure and particle size distribution of the prepared samples were examined by X-ray diffraction (XRD) and laser particle size analyzer, respectively. The results show that Sample B has more crystalline defects and smaller average diameter than Sample A. The cyclic voltammetry and electrochemical impedance spectroscopy measurements indicate that Sample B has better electrochemical performance than Sample A, such as better reaction reversibility, lower charge-transfer resistance and better cyclic stability. Proton diffusion coefficient of Sample B is 1.96×10-10cm2/s, which is two times as large as that (9.78×10-11cm2/s) of Sample A. The charge-discharge tests show that the discharge capacity (308 mA·h/g) of Sample B is 25 mA·h/g higher than that of Sample A (283 mA·h/g).
Key words: Al/Co co-doped α-Ni(OH)2; ultrasonic co-precipitation method; proton diffusion coefficient; charge-transfer resistance; electrochemical performance
1 Introduction
Nickel hydroxide has been extensively used as an active material of the positive electrode in rechargeable alkaline batteries. There are two phases known as α and β, which are transformed to γ-NiOOH and β-NiOOH, respectively during charging [1]. The β-Ni(OH)2 only has a theoretical capacity of 289 mA·h/g and is easily transformed to γ-NiOOH when overcharge happens [2], the γ-NiOOH can lead to a subsequent swelling of the positive electrode. While, α-Ni(OH)2 has been attracted much attention since the α-Ni(OH)2 not only has a superior theoretical capacity (482 mA·h/g), but also avoids the volume expansion in the charge-discharge process due to the similar lattices of α-Ni(OH)2 and γ-NiOOH. Unfortunately, α-Ni(OH)2 is easily converted to β-Ni(OH)2 in alkaline media. Many studies have been carried out through partial substitution of nickel ion by other metal ions such as Al [3], Co [4], and Zn [5] in order to improve the stability of α-Ni(OH)2. Although the stable α phase structure can be obtained after adding metal ions in the Ni(OH)2, the performance of Ni-MH batteries is adversely affected, such as the capacity fading, the internal resistance increasing and cycle life decreasing during charge-discharge process.
Also, it is difficult to synthesize nano-sized α-Ni(OH)2 because of its agglomeration. Thus, the application of α-Ni(OH)2 is limited in Ni-MH batteries. Chemical co-precipitation is the commonly used method to prepare α-Ni(OH)2, but the powder shows coarse aggregates. Based on the above consideration, in the present study, nano-sized α-Ni(OH)2 was synthesized by ultrasonic co-precipitation method, which can produce discrete and uniform particles due to the dispersive action of ultrasonic.
2 Experimental
2.1 Preparation of nickel hydroxide
The Sample B was prepared as follows. A mixed solution containing NiCl2·6H2O, Al2(SO4)3·18H2O, Co(NO3)2·6H2O (the molar ratio of Ni:Al:Co is 1:0.10:0.17) and the proper amount of sodium hydroxide aqueous solution were dropped into a mother liquid synchronously while stirring at 50 °C. Sodium hydroxide was modulated to keep the pH value at 9.00±0.10. The agitation lasted 5 h after the dropping was finished, and ultrasonic was applied in the whole processes. The suspension was aged for 12 h, and then washed three times with distilled water and twice with anhydrous ethanol. The attained nickel hydroxide product was dried at 80 °C. The same procedure was carried out to synthesize Sample A except no application of ultrasonic.
2.2 Preparation of nickel hydroxide electrode
The pasted nickel electrodes were prepared as follows. 8% Samples A or B, 74% commercial micro-size spherical nickel, 15% nickel powder, 2% CMC were mixed thoroughly with 1% PTFE solution. The paste obtained was incorporated into nickel foam substrates with the geometrical size of 2.5 cm×2.5 cm. The pasted electrodes were dried naturally and then mechanically pressed. A slurry containing 88% AB5-type metal hydride powder, 2% CMC as a binder and 10% nickel powder was forced into the same nickel substrates, then dried and compressed to obtain negative electrodes.
2.3 Physical characterization
The structures of the samples were determined using X-ray diffraction (XRD) (D/max-ⅢA X-ray diffractometer, Rigaku, Japan) with Cu Kα radiation (λ=1.54 ?) operated at 36 kV and 20 mA. Particle size distribution (PSD) was analyzed using a Nanotrac 150 particle size analyzer.
2.4 Electrochemical measurements
Electrochemical tests are performed in a three-compartment electrolysis cell at ambient temperature. The cell comprised a working electrode (the positive electrode), a counter electrode (the negative electrode) and an Hg/HgO reference electrode. Electrolyte of 7 mol/L KOH and 0.05 mol/L LiOH was used. Both cyclic voltammetry (CV) and electrochemical impedance measurements (EIS) were conducted by using a Chenhua CHI7600 model electrochemical workstation. The scanning rate of CV test ranges from 0.02 V/s to 0.1 V/s and the cell potential ranges from -0.2 to 0.7 V. EIS measurements were made at open circuit potential in the frequency range of 10 kHz-100 Hz.
Negative electrodes (the cathode) and positive electrodes (the anode) were assembled with polypropylene as the separator to form simulated batteries. Galvanostatic charge-discharge tests were conducted at 0.2C rate to cut-off voltage of 1.0 V by using a Neware BTS-51800 battery testing system at ambient temperature.
3 Results and discussion
3.1 Characterization of nickel hydroxide samples
The particle size distribution for the prepared nickel hydroxides is shown in Fig. 1. It can be seen that the size is uniformly distributed for both samples, but the average diameter of Sample A (90 nm) is bigger than that (61 nm) of Sample B. It may be due to some degree of agglomeration without ultrasonic. This can be understood that high temperature and high pressure will be generated from the cavitation when the ultrasonic is used in the preparation of the samples. The energy provided by the ultrasonic will cause the rate of nucleation to enhance several orders [6], which results in forming ultrafine particles. The cavitation also produces a lot of tiny bubbles on the surface of solid particles, and thus greatly decreases the specific surface free energy of particles in order to constrain the growth and agglomeration of crystal nucleus. Meanwhile, ultrasonic cavitation will produce the shock-wave and internal jet, which can break the agglomerates into pieces, and lead to small particle size.
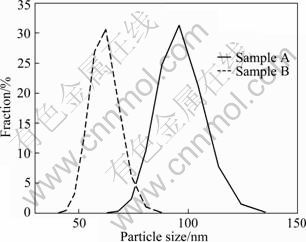
Fig. 1 Particle size distribution of Samples A and B
The XRD patterns of both samples given in Fig. 2 are similar to the standard XRD pattern of α-Ni(OH)2·0.75H2O (JCPDS 38-0715). The asymmetric diffraction peak located in the range of 32°-36° is the characteristic of the turbostratic disorder in α-Ni(OH)2 [7]. The peaks corresponding to all the reflections in the XRD patterns of Sample B are slightly broadened as compared with those in the patterns of Sample A. Moreover, the diffraction peak of Sample B corresponding to crystal reflections (015) and (018) cannot be obviously distinguished, while other peaks appear at the same positions. This illustrates that the α-Ni(OH)2 prepared by ultrasonic co-precipitation method has lower crystallinity and smaller particle size than those obtained by co-precipitation method, as shown in Fig. 1. The proper broadening of reflections caused by smaller size may increase the structural defects [8-9]. Thus, more structural defects are formed in the Sample B, which is beneficial to improving the electrochemical performance for Ni(OH)2 due to the presence of disorders [10].
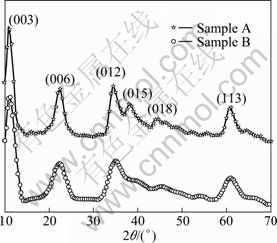
Fig. 2 XRD patterns for Samples A and B
3.2 Cyclic voltammetric behavior
Figure 3 shows the typical cyclic voltammograms of two Ni(OH) 2 at scanning rate of 0.1 V/s. The anodic peaks are due to the oxidation of α-Ni(OH)2 to γ-NiOOH [11], similarly the cathodic peaks are for the reverse process. The cyclic voltammetric parameters corresponding to Fig. 3 are listed in Table 1. The ΔEO,R is taken as an estimate of the reversibility of the redox reaction, the smaller ΔEO,R is, the more reversible the electrochemical reaction is [12]. Compared with ΔEO,R value (0.324 V) of sample A, ΔEO,R value (0.301 V) of Sample B decreases, suggesting that the ultrasonic can play a positive role in improving the reversibility of electrode reaction. The oxygen evolution reaction (OER) is known as a parasitic reaction during charging of nickel hydroxide. The separation between the oxidation peak potential and the OER potential (EOER-EO) is very important, the large value is expected [13]. From Table 1, the EOER-EO of Sample B is larger than that of Sample A, thus more active materials can be utilized during charge and discharge, which is in accordance with the charge-discharge results.
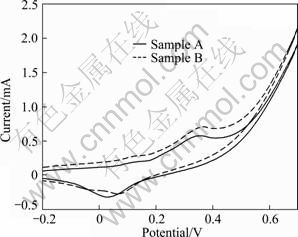
Fig. 3 Cyclic voltammograms of Samples A and B at scanning rate of 0.1 V/s
Table 1 CV results of Samples A and B
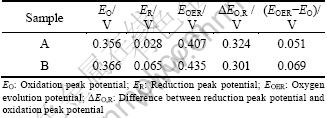
It is well known that the proton diffusion process controls the rate of battery reaction [14]. Therefore, it is of great importance to study the proton diffusion coefficient. In case of semi-infinite diffusion, at 25 °C, the peak current may be expressed by the classical Randle-Sevick equation [15]:
(1)
where Ip is the anodic peak current; n is the electronic number of reaction (the value is about 1 for Ni(OH)2); S is the real surface area of the electrode; D is the diffusion coefficient; v is the scanning rate; C0 is the initial concentration of active material. For nickel hydroxide electrode:
(2)
where ρ is the theoretical density of Ni(OH)2 (2.82 g/cm3); M is the mole mass of Ni(OH)2 (92.7 g/mol); x is the molar fraction of sample powders in composite electrode.
Cyclic voltammograms for Samples A and B under various scanning rates are presented in Fig. 4. As the scanning rate increases, the anodic peak potential shifts to more positive direction, while the cathodic peak only has a slight movement toward the negative direction. This result is similar to that reported previously [16-18]. The linear relationship between Ip and v1/2 for both samples can be founded, as shown in Fig. 5. From the slope of the fitted line in Fig. 5 and Eq. (1) we can get the proton diffusion coefficient D. Proton diffusion coefficient of sample A is 9.78×10-11 cm2/s, and that of Sample B is 1.96×10-10 cm2/s. This can be explained by the fact that the sample B has a smaller particle size and large specific surface area, which not only provides more chances for the particles to contact the electrolyte solution, but also shortens the diffusion distance in solid-state. Moreover, the intercalation and release of protons into and from the nickel hydroxide host lattice can be enhanced by crystal imperfection.
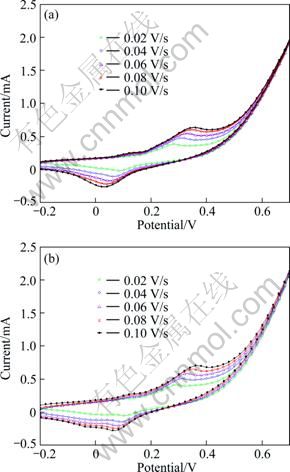
Fig. 4 Cyclic voltammograms for Samples A (a) and B (b) under different scanning rates
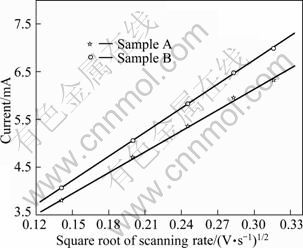
Fig. 5 Relationship between anodic peak current and square root of scanning rate for Samples A and B
3.3 Electrochemical impedance spectroscopy measurement
Figure 6 shows the Nyquist plots for both electrodes at steady state. The corresponding equivalent circuit for impedance analysis is displayed as the inset of Fig. 6, where RL is the resistance of the solution; Rct is the charge-transfer resistance; ZW is the Warburg resistance; and QC is the constant phase element for the double layer capacitance. For both electrodes at high frequencies (HF), the semicircle is characteristic of the charge-transfer resistance acting in parallel with the double layer capacitance [19]. At low frequencies (LF), a straight line having an angle of 45° with the real axis indicates a linear Warburg portion, being characteristic of the semi-infinite diffusion. It is worth noting that the line having a slope higher than 45° is not discovered at LF, which may result from the disappearance of finite diffusion effects and is related to the finite length [20]. Generally, the electrode reaction occurring at nickel hydroxide is controlled by charge transfer and proton diffusion. The simulated values of the elements for the equivalent circuits are listed in Table 2. From Table 2, Sample B has smaller Rct than Sample A, but the values of QC are converse, which may be due to the fact that the Sample B has a large efficient active specific surface area for the electrochemical reactions because of the smaller sized crystals and incorporating with relatively more foreign ions and water molecules [21]. The results illustrate that the Sample B exhibits a lower resistance to charge-transfer and higher efficient proton diffusion than that of Sample A during the electrochemical reaction.
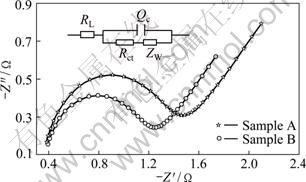
Fig. 6 Nyquist plots and equivalent circuit of Samples A and B
Table 2 Impedance parameters for Samples A and B
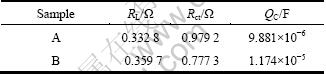
3.4 Galvanostatic charge-discharge measurement
Figure 7 shows the charge-discharge curves of two electrodes at 0.2C rate. It can be seen that the discharge capacity of Sample B is 308 mA·h/g, which is 25 mA·h/g higher than that (283 mA·h/g) of Sample A. Moreover, Sample B has a lower charge potential and higher discharge potential compared with Sample A, which is in good agreement with the CV tests. The cyclic behavior of the two electrodes at 0.2C rate is illustrated in Fig. 8. Two curves show that discharge capacity increases firstly, then it reduces after reaching the maximum value. However, the discharge capacity of sample A decreases sharply after 10 cycles, and just has a slight decrease for Sample B. The deterioration rates Rd [22] for Samples A and B are 4% and 23%, respectively. This indicates that Sample B has much better cyclic stability than sample A during the cycle process.
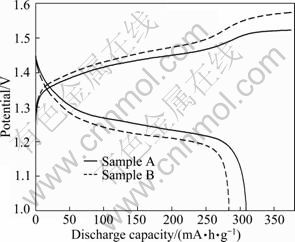
Fig. 7 Charge-discharge curves of Samples A and B at 0.2C rate
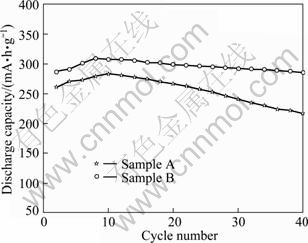
Fig. 8 Cyclic performance of Samples A and B at 0.2C rate
4 Conclusions
1) Al/Co co-doped α-Ni(OH)2 prepared by ultrasonic co-precipitation method have smaller grain size and more crystalline defects than the Al/Co co-doped α-Ni(OH)2 prepared by co-precipitation method.
2) Due to the utilization of ultrasonic, sample B has a relatively higher proton conductivity. The electrode reaction is found to be controlled by proton diffusion and the proton diffusion coefficient is 1.96×10-10cm2/s for Sample B and 9.78×10-11cm2/s for Sample A.
3) Electrochemical impedance spectroscopy measurements show that the sample prepared by ultrasonic co-precipitation method has a lower resistance and higher double layer capacitance than the sample prepared by co-precipitation method.
4) The galvanostatic charge-discharge experiment results show that the discharge capacity of Sample B reaches 308 mA·h/g, while that of Sample A is 283 mA·h/g. The utilization of ultrasonic can increase the discharge potential and decrease the charge potential. The cycle stability of Sample B is obviously improved compared with that of Sample A.
References
[1] WANG Hong, TANG Zhi-yuan, LIU Yuan-gang, LEE Chang-sheng. Synthesis and behavior of Al-stabilized α-Ni(OH)2 [J]. Transactions of Nonferrous Metals Society of China, 2009, 19(1): 170-175.
[2] JAYASHREE R S, VISHNU, KAMATH P. Layered double hydroxides of Ni with Cr and Mn as candidate electrode materials for alkaline secondary cells [J]. J Power Sources, 2002, 107(1): 120-124.
[3] INDRIN L, DIXIT M, KAMATH P V. Electrosynthesis of layered double hydroxide of nickel hydroxide of nickel with trivalent cations [J]. J Power Sources, 1994, 52: 93-97.
[4] FAURE C, DELMAS C, WILLMANN P. Electrochemical behavior of cobalt nickel hydroxide electrodes [J]. J Power Sources, 1991, 36: 497-506.
[5] TESSIER C, DEMOURGUES-GRERLOU L, FAURE C. Structural and textural evolution of zinc substituted nickel hydroxide electrode materials upon ageing in KOH and upon redox cycling [J]. Solid State Ionics, 2000, 133(1-2): 11-23.
[6] SUSLICK K S, CASADONATE D J , GREEN M L H, THOMPSON M E. Effects of high intensity ultrasound in inorganic solids [J]. Ultrasonics, 1986, 25: 56-59.
[7] XIAO H M, WANG J M. Influence of Al content on the structure and electrochemical performance of Al-substituted α-Ni(OH)2 [J]. Journal of Inorganic Materials, 2004, 19(3): 463-470.
[8] TESSIER C, HAUMESSERP H, BERNARD P, DELMAS C. The structure of Ni(OH)(2): From the ideal material to the electrochemically active one [J]. J Electrochem Soc, 1999, 146(6): 2059-2067.
[9] KOHLER U, ANTONIUS C, BAUERLEIN P. Advances in alkaline batteries [J]. J Power Sources, 2004, 127: 45-52.
[10] JAYASHREE R S, KAMATH P V, SUBBANNA G N. The effect of crystallinity on the reversible discharge capacity of nickel hydroxide [J]. J Electrochem Soc, 2000, 147(6): 2029-2032.
[11] FAURE C, DELMAS C, FOUASSIER M. Characterization of a turbostratic nickel hydroxide quantitatively obtained from an Ni2SO4 solution [J]. J Power Sources, 1991, 35: 279-290.
[12] XIE S Y, FANG Q, CHENG Y, JIAN X Y, ZHU L, WANG Z, YU H. Effects of erbium added by mechanically mixture and surface coating on high temperature performances of nickel hydroxide electrode [J]. Journal of the Chinese Rare Earth Society, 2008, 26(5): 570-576.
[13] ZHANG Y S, CAO X Y, YUAN H T, ZHANG W H, ZHOU Z X. Oxygen evolution reaction on Ni hydroxide film electrode containing various content of Co [J]. Int J Hydrogen Energy, 1999, 24: 529-536.
[14] ZIMMERMAN A H, EFFA P K. Discharge kinetics of the nickel electrode [J]. J Alloys Compd, 1984, 131(4): 709-713.
[15] Southampton Electrochemistry Group. Instrumental methods in electrochemistry [M]. England: Ellis Horwood, 1985.
[16] QI J Y, XU P, LV Z S, LIU X R, WEN A H. Effect of crystallinity on the electrochemical performance of nanometer Al-stabilized α-nickel hydroxide [J]. J Alloys Compd, 2008, 462: 164-169.
[17] YI Q F, ZHANG J J, HUANG W, LIU X P. Electrocatalytic oxidation of cyclohexanol on a nickel oxyhydroxide modified nickel electrode in alkaline solutions [J]. Catalysis Communications, 2007, 8: 1017-1022.
[18] ZHAI H J, WANG X Y, YANG H P, LI J M. Studies on the electrode characteristics of metal-substituted α-Ni(OH)2 [J]. Chin J Power Sources, 2003, 27: 197-200.
[19] DAI J X, LI S F Y, XIAO T D, WANG D M, REISNER D E. Structural stability of aluminum stabilized alpha nickel hydroxide as a positive electrode material for alkaline secondary batteries [J]. Journal of Power Sources, 2000, 89: 40-45.
[20] LIU B, YUAN H T, ZHANG Y S. Impedance of Al-substituted alpha-nickel hydroxide electrodes [J]. Int J Hydrogen Energy, 2004, 9(5): 453-458.
[21] ZHOUH B, ZHOU Z T. Preparation, structure and electrochemical performances of nano-sized cathode active material Ni(OH)2 [J]. Solid State Ion, 2005, 176: 1909-1914.
[22] ZHAO Y L, WANG J M, CHEN H, PAN T, ZHANG J Q, CAO C N. Al-substituted alpha-nickel hydroxide prepared by homogeneous precipitation method with urea [J]. Int J Hydrogen Energy, 2004, 9(8): 889-896.
超声波对α-Ni(OH)2电极结构及电化学性能的影响
张仲举, 朱燕娟, 包 杰, 周卓均, 叶贤聪, 许庆盛
广东工业大学 物理与光电工程学院,广州 510006
摘 要:分别采用共沉淀法和超声波共沉淀法制备Al/Co复合掺杂α-Ni(OH)2样品A和B,用XRD和激光粒度仪表征样品的晶相结构和粒度分布。结果表明,样品B比样品A具有较多的晶格缺陷和较小的平均粒径。循环伏安特性及电化学阻抗谱测试显示,样品B比样品A具有更好的电化学性能:较好的反应可逆性、较低的电荷转移电阻和较高的循环寿命等。样品B的质子扩散系数为1.96×10-10cm2/s,约为样品A(9.78×10-11cm2/s)的2倍。充放电测试显示,样品B的放电比容量达到308 mA·h/g,比样品A的放电比容量高25 mA·h/g。
关键词:Al/Co复合掺杂α-Ni(OH)2;超声波共沉淀法;质子扩散系数;电荷转移电阻;电化学性能
(Edited by LI Xiang-qun)
Foundation item: Project (10774030) supported by the National Natural Science Foundation of China; Project (2008J1-C161) supported by the Science and Technology Program of Guangzhou City of China
Corresponding author: ZHU Yan-juan; Tel: +86-13610281143; E-mail: Yanjuanzhu007 @126.com
DOI: 10.1016/S1003-6326(11)61106-0