J. Cent. South Univ. Technol. (2011) 18: 1877-1882
DOI: 10.1007/s11771-011-0917-x
Preparation of nano-structured Ag solid materials and
application to surface-enhanced Raman scattering
YI Zao(易早)1, 2, CHEN Yan(陈艳)1, 2, CHEN Shan-jun(陈善俊)1, 3, TAN Xiu-lan(谭秀兰)1,
NIU Gao(牛高)1, LUO Jiang-shan(罗江山)1, TANG Yong-jian(唐永建)1, 3, YI You-gen(易有根)2
1. Research Center of Laser Fusion, China Academy of Engineering Physics, Mianyang 621900, China;
2. School of Physical Science and Technology, Central South University, Changsha 410083, China;
3. Institute of Atomic and Molecular Physics, Sichuan University, Chengdu 610065, China
? Central South University Press and Springer-Verlag Berlin Heidelberg 2011
Abstract: Silver nano-particles with average diameter of about 60 nm were compacted in a high-strength mold under different pressures at 523 K to produce nano-structured Ag solid materials. The structure and characteristic of the nano-structured Ag solid materials (NSS-Ag) were studied using X-ray diffraction (XRD), scanning electron microscope (SEM) and Raman spectrometer. The NSS-Ag could be used as highly efficient surface-enhanced Raman scattering (SERS) active substrates. The common probe molecules Rhodamine 6G (R6G, 1×10-10 mol/L) were used to test the SERS activity on these substrates at very low concentrations. It is found that the SERS enhancement ability is dependent on the density of NSS-Ag. When the relative density of NSS-Ag is 83.87%, the materials reveal great SERS signal.
Key words: nano-structured Ag solid material; flow-levitation method; relative density; Rhodamine 6G; surface-enhanced Raman scattering
1 Introduction
Surface-enhanced Raman scattering (SERS) is an effective analytical tool for the detection of molecules adsorbed on metal surfaces because of its high sensitivity and molecular detection capabilities [1-5]. Recently, many different methods have been employed to fabricate SERS substrates with various surface roughness features, including femtosecond laser technology [6], electrochemically roughened electrodes [7-8] and metal colloidal suspensions [9-10]. However, the development of a SERS-based surface chemical analysis application requires an effective SERS-active substrate that not only can provide strong enhancement factor and great reproducibility, but also is facile and relatively inexpensive to fabricate and store.
Silver nano-particles prepared by the flow-levitation method (FL) [11] which have high purity for the heating system are non-touching. The average grain size of the nano-particles prepared by FL technique can be controlled easily through the adjustment of the process parameters. Nano-particles become nano-structured bulk easily using vacuum-warm-compaction (VWC) technique [12]. The VWC technique can make inert gases generate desorption and accelerate atoms diffusion, so it is an improvement of flow-levitation-cold- compaction method (FLCC) [13]. Its process is shown as: metal nano-powders are prepared by FL technique→ metal nano-powders are loaded on glove box→metal nano-structured bulks are pressed by VWC technique→ nano-structured solid materials are obtained.
In this work, a novel SERS-active substrate was synthesized. The NSS-Ag samples were made using FL method and VWC technique. The structure and SERS activity of NSS-Ag samples were characterized by SEM, XRD and SERS measurement, respectively. It is found that the SERS enhancement ability is dependent on the relative density of NSS-Ag samples. These SERS active substrates show high SERS enhancement ability by adsorbing R6G molecules (1×10-10 mol/L). The study focuses on the relationship between SERS enhancement factor (G) and the different relative densities of NSS-Ag samples.
2 Experimental
2.1 Preparation of SERS-active substrates
Nano-structured Ag particles were prepared by FL method [11] and then filled into the high strength mold in ZKSTX-1 type vacuum-glove-chest with Ar (purity> 99.99%, volume fraction) protection. After the mold was put into the chamber of ZM-18-10Y type vacuum- hot-press, the chamber was pumped to a pressure of 0.005 MPa, and then the mold with nano-structured Ag particles was heated for 30 min in order to release absorbed gas prior to pressing. Subsequently, the nano-structured Ag particles were compacted at 523 K, under a uniaxial load of 0.01-1.00 GPa for duration of 20-60 min, resulting in disc-shaped samples with 10 mm in diameter and 0.5-1.00 mm in thickness.
2.2 SERS measurement
R6G solution was diluted to the concentration of 1×10-10 mol/L with methanol, and then 20 μL of the solution was dropped onto the SERS substrates. The SERS substrates were deposited in the vessel of 65%-75% humidity for 30 min in order to form the symmetrical probe adsorptive layer. The solvent of the SERS substrates was allowed to evaporate under ambient condition. As is known, SERS spectra of high-density R6G molecules were difficult to obtain because of the strong fluorescence. So, before the SERS experiments, the substrates were washed with deionized water to remove the physically adsorbed R6G molecules, and then dried in a stream of nitrogen gas.
2.3 Characterization of SERS active substrates
A Philips X’Pert Pro MPD type X-ray diffractometer (made in Netherlands, Cu Kα radiation, λ= 0.154 056 nm) was used to identify the phase of the specimens. The density of the compacted samples was measured using Archimedes’ principle. A JSM-6360LV scanning electron microscope (SEM) was employed to observe the microstructure of the as-prepared specimens. Raman spectra were obtained with a Renishaw 2000 model confocal microscopy Raman spectrometer with a CCD detector and a holographic notch filter (Renishaw Ltd, Gloucestershire, UK). The microscope attachment was based on a Leica DMLM system, and a 50× objective was used to focus the laser beam onto a spot with approximately 1 μm in diameter. Radiation of 514.5 nm wavelength from an air cooled argon ion laser (Spectra-Physics Model 163-C4260) was used for excitation. All of the spectra reported were the results of a single 20 s accumulation.
3 Results and discussion
According to the VWC technique, the relative density of NSS-Ag bulks is mainly related to the pressure and the pressure-holding-time of suppression. At the beginning of the compaction process, the increase of density is obvious with the pressure increasing. At this stage, densification is driven by the motion of particles and the filling of void among particles. When the pressure continues to increase, the nano-particles will deform. The deformation of the particles begins at the contact area of transfer pressure particles. The contact area of stress must exceed the yield limit of the material in order to ensure further denseness [13]. In the present experiment, the maximum pressure is 1.00 GPa, and the relative densities of NSS-Ag samples are listed in Table 1. The relative density is the ratio of density of NSS-Ag samples to the density of pure Ag (10.53 g/cm3). It is found that the pressure and pressure-holding-time have significant impact upon the relative density of the NSS-Ag samples.
Table 1 Relative density of NSS-Ag samples
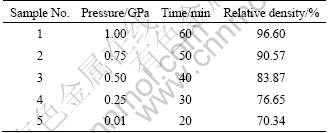
Figures 1 shows the SEM images of the products. Figure 1(d) shows the morphology of spherical Ag nano-particles. The size distribution of these nano- particles is narrow (35-85 nm). The average diameter of Ag nano-particles is 60 nm. Figures 1(a)-(c) present the SEM images of the Ag nano-particles after different pressure and pressure-holding-time. The values of the relative density of the NSS-Ag samples are 70.34%, 83.87%, 96.60%, respectively. Figures 1(a)-(c) show that some pores exist in the NSS-Ag samples. As can be seen, some gaps exist in some local areas of NSS-Ag samples when the massive particles are suppressed by the nano Ag powder with average size of about 60 nm. This may be due to the high surface activity of nano-silver powder. The nano-silver particles can not be compact contacted in the suppression process because the nano-silver particles adsorb a lot of gas. The density of NSS-Ag samples increases when the pressure of suppression increases. The mutual extrusion and friction of nano-Ag particles lead to the compact particles.
X-ray diffraction was carried out to characterize the structure of the samples and determine whether they are oxidized. Figure 2 shows the X-ray diffraction patterns of the samples with different relative density. The values of the relative density of the samples are 96.60% (Fig.2(a)), 90.57% (Fig.2(b)), 83.87% (Fig.2(c)), 76.65% (Fig.2(d)) and 70.34% (Fig.2(e)), respectively. The five patterns show the polycrystalline nature of the products with a high intensity diffraction peak at about 38.00°, corresponding to the (111) crystalline plane of silver. Some other less intense peaks are observed in these spectra at about 44.26°, 64.40° and 77.32°, corresponding to the (200), (220) and (311) crystalline planes of the cubic crystalline structure of silver. According to the X-ray diffraction theory, the grain sizes of the samples are obtained by separating the X-ray diffraction peak with multiple peaks, linearly analyzing the isolated diffraction peaks, separating the geometric width that caused by the equipment and the physical width that caused by the micro-strain physical factors, deducting the linear function of geometric width and dealing with Warren-Averbach Fourier transform analysis method. The crystal parameters are calculated to be 59 nm (1#), 61 nm (2#), 60 nm (3#), 61 nm (4#), 60 nm (5#) respectively, which are in agreement with the literature report (PCPDF NO. 04-0783). These crystal parameters basically are the same. It can be inferred that the crystal size of the single nano-powder will not be changed and new crystal will not be induced by the pressure in the present range.
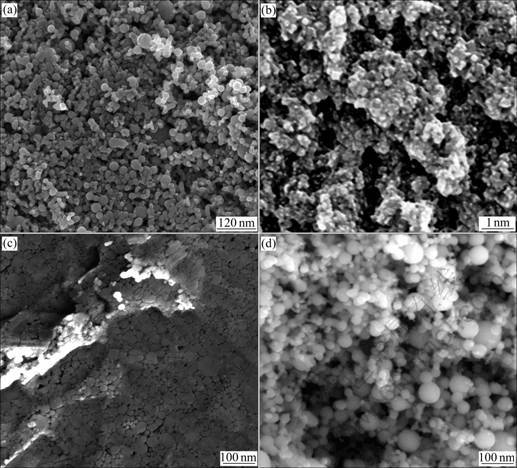
Fig.1 SEM images of NSS-Ag samples with different relative density: (a) 70.34%; (b) 83.87%; (c) 96.60%; (d) Nano-structured Ag powder
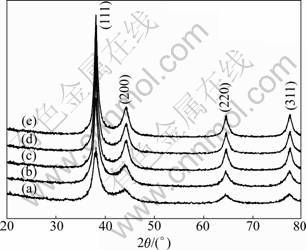
Fig.2 X-ray diffraction pattern of NSS-Ag samples with different relative density: (a) 96.60%; (b) 90.57%; (c) 83.87%; (d) 76.65%; (e) 70.34%
Rhodamine 6G (R6G) is chosen as an analyte in the present study because it has been well characterized by SERS and by resonance Raman spectroscopy. The SERS spectra of single R6G molecules have been obtained [14] and most of the prominent Raman bands have been assigned [15]. The 514.5 nm line from an argon ion laser was used in the present study. This wavelength corresponds to a resonant excitation of R6G, so the surface-enhanced resonance Raman spectrum (SERRS) is acquired. The SERRS is accepted that the increase in signal is due to the increase in the apparent cross-section of the molecules. So, when estimating the SERS enhancement factor, the non-SERS cross-section should be considered first. R6G is a dye with strong adsorption at a maximum value of 528 nm which is close to the resonance Raman scattering at 514.5 nm laser excitation. The experimental resonance Raman scattering spectrum is difficult to obtain because of the overwhelming fluorescent background at 514.5 nm. Here, the estimation of the SERS enhancement factor of R6G at 514.5 nm reported by GUPTA and WEIMER [16] was adopted as
(1)
where G is the SERS enhancement factor; NRaman and Nsurf denote the number of probe molecules which contribute to the normal and enhanced signal intensity, respectively; while IRaman and Isurf denote the corresponding normal Raman and SERS intensities. Because the R6G molecules are adsorbed into the SERS-active substrates, the SERS enhancement factor can be written in the following form:
(2)
where M is the number of R6G molecules dropped onto the normal substrate; S is the geometrical area of R6G casting film on the normal surface; A is the recorded area of the laser spot; SR6G is the effective occupied area of single R6G molecule onto the SERS-active substrate, herein, is 222 ?2. It is known that, under the same experimental conditions, the relationship of the R6G signal intensity between the normal Raman scattering and the fluorescence is shown as
(3)
where σRaman and σflu are the cross-sections of the normal Raman scattering and the fluorescence at 514.5 nm, and they are 10-25 and 10-16 cm2/sr, respectively [17]. So, it can be estimated that the IRaman dvivided by the Iflu is with an order of 10-9. Finally, G can be written as the following:
(4)
Figure 3 shows the SERS spectra of the R6G molecules adsorbed onto the pure Ag flake without Ag nano-powders and Ag nano-powders deposited onto the pure Ag flake. Figure 3(a) shows the resonance Raman scattering spectrum of the R6G deposited film that is prepared by dropping 20 μL R6G methanol solution (10-5 mol/L) onto a clean pure Ag flak. As expected, no signal can be distinguished. Figure 3(b) shows the Raman band of the 20 μL R6G methanol solution (1×10-10 mol/L) deposited onto Ag nano-powders. Several strong bands at 1 649, 1 574, 1 506, 1 361, 1 310, 1 186, 773 and 610 cm-1 are observed on the substrate. They are assigned to xanthenes ring stretch, ethylamine group wag and carbon—oxygen stretch of R6G, respectively [18]. Many authors have discussed the SERS of R6G molecules onto the silver surface. In early 1980s, HILDEBRANDT and STOCKBURGER [19] studied the SERS spectra of R6G on colloidal silver and pointed out that the addition of anion could improve the detection limit of R6G on silver colloid. In their experiment, when the Cl- ions were added to silver colloid, the detection limit was found to reach 10-9 mol/L while it was 10-6 mol/L without additional Cl-. Evidently the anion has very strong effect on the SERS spectra of R6G on silver colloid. However, in the present experiment, the SERS spectra of R6G at the concentration of 10-10 mol/L without additional anion and any other electrolytes were obtained. The main vibration band of R6G is distinct on the SERS spectra.
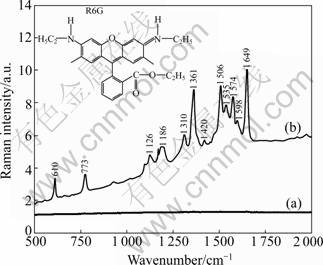
Fig.3 SERS spectra of Rhodamine 6G adsorbed on pure Ag flake with no Ag nano-powders (a) and Ag nano-powders deposited onto pure Ag flake (b) (Laser power (514.5 nm) on samples is about 40 μW)
As is known, the shape and the aggregate of particles are important parameters for the surface enhancement in terms of the electromagnetic theory of SERS. From the SEM results, it is known that the shapes of the NSS-Ag samples are different. Figure 4 shows the SERS spectra of the R6G molecules adsorbed onto the different relative densities of NSS-Ag samples. On these SERS-active substrates, a low concentration of R6G produces a clear enhanced effect at 1 649 cm-1, which is one of the main characteristic bands (Fig.4). An obvious trend is that the enhancement ability increases strongly with the change of the relative density of NSS-Ag samples and it will achieve the maximum enhancement when the relative density of NSS-Ag is 83.87%. The signal of SERS will decrease to approach the signal of pure Ag solid material when the density of NSS-Ag samples increased. When the pressure decreases, the signal of SERS will decrease because the number of “junctions” decreases and thus cannot provide a more intense SERS enhancement. As the size of the aggregates increases, the number of “junctions” increases and thus provides a more intense SERS enhancement [20]. In conclusion, under the same experimental conditions (integration time: 20 s, power: 1%), NSS-Ag particles reveal a greater SERS signal when the relative density of NSS-Ag material is 83.87%.
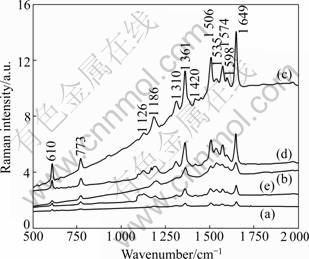
Fig.4 SERS spectra of Rhodamine 6G (1×10-10 mol/L) adsorbed on different relative densities of NSS-Ag materials (Laser power (514.5 nm) on samples is about 40 μW): (a) 96.60%; (b) 90.57%; (c) 83.87%; (d) 76.65%; (e) 70.34%
According to Eqs.(1)-(4), the SERS enhancement factor (G) can be calculated when the SERS strong bands are at 1 649, 1 506, 1 361, 1 186 and 773 cm-1, respectively. Figure 5 shows the relationship of SERS enhancement factor (G) and the different relative densities of NSS-Ag samples. The SERS signal intensity at 1 649 cm-1 is 269.4 (the relative density is 83.87%), 238.3 (the relative density is 76.65%), 201.4 (the relative density is 90.57%), 120.5 (the relative density is 70.34%), 80.3 (the relative density is 96.60%), respectively. Figure 5 shows that G reaches the strongest value when the relative density of NSS-Ag material is 83.87%.
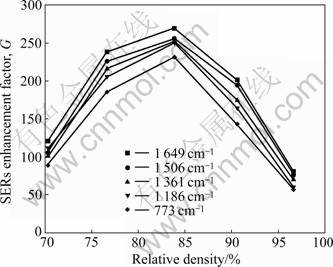
Fig.5 Relationship of SERS enhancement factor (G) and different relative densities of NSS-Ag samples
4 Conclusions
1) A novel method to prepare SERS-active substrates is presented, which shows great SERS enhancement ability tested by R6G. Ag nano-particles with average diameter of 60 nm are synthesized using FL method. NSS-Ag of 10 mm in diameter and 0.5- 1.00 mm in thickness is made using VWC technique. The relative density of NSS-Ag can be adjusted by changing the pressure of suppression and the pressure- holding-time. The crystal size of a single nano-powder will not be changed and the new crystal will not be induced by the pressure in the present range.
2) The SERS active substrates show high SERS enhancement ability by adsorbing R6G molecules (1×10-10 mol/L). When the relative density of NSS-Ag is 83.87%, the material reveals a great SERS signal. The synthesizing way of SERS-active substrates can be applied to other metal nano-particles. The high SERS enhancement ability and good stability of these new substrates will hold great promise in ultrasensitive detection and bioassays that are free from photo- denaturation and undesirable heat effects.
References
[1] ZHANG Z L, YIN Y F, JIANG J W, MOET Y J. Single molecule detection of 4-dimethylaminoazobenzene by surface-enhanced Raman spectroscopy [J]. Journal of Molecular Structure, 2009, 920(1/2/3): 297-300.
[2] OATES T W H, SUGIME H, NODA S. Combinatorial surface- enhanced Raman spectroscopy and spectroscopic ellipsometry of silver island films [J]. J Phys Chem C, 2009, 113(12): 4820-4828.
[3] DENIS P, TAN S L, MELEK E, HENRY D, SVETLANA S. In situ SERS study of Rhodamine 6G adsorbed on individually immobilized Ag nanoparticles [J]. Journal of Raman Spectroscopy, 2006, 37(7): 762-770.
[4] JEONG M B, SEUNG J L, MARTIN M. Polarized surface-enhanced Raman spectroscopy from molecules adsorbed in nano-gaps produced by electromigration in silver nanowires [J]. Nano Lett, 2009, 9(2): 672-676.
[5] JAMES A H, SILVIA P C, HIDEHO O, HIROSHI F, JOHAN H, HIROSHI U. Subdiffraction limited, remote excitation of surface enhanced Raman scattering[J]. Nano Lett, 2009, 9(3): 995-1001.
[6] ERIC D D, NATHAN H M, STEPHEN K D, ERIC M. Femtosecond laser-nanostructured substrates for surface-enhanced Raman scattering [J]. Langmuir, 2009, 25(3): 1790-1794.
[7] LIU G Q, CAI W P, LIANG C H. Trapeziform Ag nanosheet arrays induced by electrochemical deposition on Au-coated substrate [J]. Cryst Growth Des, 2008, 8(6): 2748-2752.
[8] CHAO Y W, ZHOU Q, LI Y, YAN Y R, WU Y, ZHENG J W. Potential dependent surface-enhanced Raman scattering of 4-mercaptopyridine on electrochemically roughened silver electrodes [J]. J Phys Chem C, 2007, 111(45): 16990-16995.
[9] SUN L L, SONG Y H, WANG L, GUO C L, SUN Y J, LIU Z L, LI Z. Ethanol-induced formation of silver nanoparticle aggregates for highly active SERS substrates and application in DNA detection [J]. J Phys Chem C, 2008, 112(5): 1415-1422.
[10] TANTRA R, BROWN R J C, MILTON M J T. Strategy to improve the reproducibility of colloidal SERS [J]. Journal of Raman Spectroscopy, 2007, 38(11): 1469-1479.
[11] LI C M, LEII H, TANG Y J, LUO J S, LIU W, CHEN Z M. Production of copper nanoparticles by the flow-levitation method [J]. Nanotechnology, 2004, 15(4): 1866-1869.
[12] CHU G, LIU W, TANG Y J, YANG T Z. Properties of nanocrystalline copper prepared by vacuum-warm-compaction method [J]. Trans Nonferrous Met Soc China, 2009, 19(2): 394-398.
[13] LIU W, YANG T Z, TANG Y J, CHU G, LUO J S. Synthesis and properties of nanocrystalline nonferrous metals prepared by flow-levitation- molding method [J]. Trans Nonferrous Met Soc China, 2007, 17(4): 1347-1351.
[14] GROCHALA W, KUDELSKI A, BUKOWSKA J. Anion-induced charge-transfer enhancement in SEES and SERRS spectra of rhodamine 6G on a silver electrode: How important is it? [J]. J Raman Spectrosc, 1998, 29(8): 681-685.
[15] KEATING C D, KOVALESKI K K, NATAN M J. Heightened electromagnetic fields between metal nanoparticles: Surface enhanced Raman scattering from metal-cytochrome c-metal sandwiches [J]. J Phys Chem B, 1998, 102(8): 9414-9425.
[16] GUPTA R, WEIMER W A. High enhancement factor gold films for surface enhanced Raman spectroscopy [J]. Chem Phys Lett, 2003, 374(3): 302-306.
[17] NIE S, EMORY S R. Probing single molecules and single nanoparticles by surface-enhanced Raman scattering [J]. Science, 1997, 275(4): 1102-1106.
[18] WEI G, ZHOU H L, LIU Z G, LI Z. A simple method for the preparation of ultrahigh sensitivity surface enhanced Raman scattering (SERS) active substrate [J]. Applied Surface Science, 2005, 240(1): 260-267.
[19] HILDEBRANDT P, STOCKBURGER M. Surface-enhanced resonance Raman-spectroscopy of rhodamine-6g adsorbed on colloidal silver [J]. J Phys Chem, 1984, 88(6): 5935-5939.
[20] WILLIAM E D, NIE S. Single-molecule and single-nanoparticle SERS: Examining the roles of surface active sites and chemical enhancement [J]. J Phys Chem B, 2002, 106(1): 311-317.
(Edited by HE Yun-bin)
Foundation item: Project(10804101) supported by the National Natural Science Foundation of China; Project(2007CB815102) supported by the National Basic Research Program of China; Project(2007B08007) supported by the Science and Technology Development Foundation of Chinese Academy of Engineering Physics
Received date: 2010-07-26; Accepted date: 2011-03-24
Corresponding author: YI You-gen, Professor, PhD; Tel: +86-816-2484233; E-mail: myyz1984@yahoo.cn