J. Cent. South Univ. (2016) 23: 122-129
DOI: 10.1007/s11771-016-3055-7

Effect of inlet temperature and equivalence ratio on HCCI engine performance fuelled with ethanol: Numerical investigation
Alireza Rahbari
Department of Mechanical Engineering, Shahid Rajaee Teacher Training University (SRTTU), Tehran, Iran
Central South University Press and Springer-Verlag Berlin Heidelberg 2016
Abstract: A numerical model is presented to investigate the performance of homogeneous charge compression ignition (HCCI) engines fueled with ethanol. Two approaches are studied. On one hand, two-step reaction mechanisms with Arrhenius reaction rates are implemented in combustion chemistry modeling. On the other hand, a reduced mechanism containing important reactions of ethanol involving heat release rate and reaction rates compatible with experimental data is employed. Since controls of combustion phenomenon and ignition timing are the main issues of these engines, the effects of inlet temperature and equivalence ratio as the controlling factors on the operating parameters such as ignition timing, burn duration, in-cylinder temperature and pressure of HCCI engines are explored. The results show that the maximum predicted pressures for thermodynamic model are about 71.3×105 Pa and 79.79×105 Pa, and for chemical kinetic model, they are about 71.48×105 Pa and 78.123×105 Pa, fairly comparable with corresponding experimental values of 72×105 Pa and 78.7×105 Pa. It is observed that increasing the initial temperature advances the ignition timing, decreases the burn duration and increases the peak temperature and pressure. Moreover, the maximum temperature and pressure are associated with richer mixtures.
Key words: HCCI engine; ethanol; two-step reaction mechanism model; chemical kinetic model
1 Introduction
The environmental impact of automotive transport systems and the need for reducing petroleum dependence lead to the development of alternative combustion strategies and the use of renewable fuels [1]. The new combustion concept for internal combustion engines namely homogeneous charge compression ignition (HCCI) combustion has been drawing the considerable attention due to high efficiency and lower nitrogen oxide (NOx) and particulate matter (PM) emissions [2]. HCCI engines have been shown to operate with a range of fuels including ethanol [3–4]. HCCI engine with ethanol as a renewable fuel produced from non-petroleum based products offers a promising solution to tackle the concerns about the effects of fossil fuel usage on global warming.
In this regard, MACK et al [5] experimentally and numerically researched the effect of diethyl ether and ethanol on the combustion properties of the HCCI engines. YAP and MEGARITIS [6] applied forced induction to bioethanol HCCI operation on a gasoline type engine, in conjunction with residual gas trapping. This retained the much reduced external heating benefits associated with residual gas trapping. In another research [7], they explored two different approaches to achieve HCCI with bioethanol, namely, trapping of internal residual gas and intake temperature heating with a high compression ratio. MEGARITIS et al [8] investigated the effect of water blending on bioethanol HCCI combustion with forced induction and residual gas trapping.
MACK et al [9] investigated an HCCI engine fueled with wet ethanol and determined the effect of the ethanol-water fraction on the engine’s operating limits, intake temperatures, heat release rates, and exhaust emissions for the engine operating with different ethanol- in-water mixtures. KOMNINOS and RAKOPOULOS [10] compared the detailed and reduced ethanol oxidation mechanism in the HCCI engine combustion using a multi-zone model. It was observed that the reduced model decreased the simulation time by 50% and the two mechanisms produced almost identical results as regards ignition timing and pollutant emissions. KHALIQ et al [11] proposed a numerical approach for a HCCI engine fuelled with wet ethanol to investigate the effects of turbocharger compressor ratio, ambient temperature, and compressor adiabatic efficiency on first law efficiency, second law efficiency and exergy destruction.
VIGGIANO and MAGI [12] presented a multidimensional numerical approach coupled with a kinetic reaction mechanism for ethanol oxidation and NOx formation for HCCI combustion in order to clarify the role of specific technical solutions, such as power boosting, downsizing, swirl motion, and the thermo- physical properties of ethanol on emissions and performance of the engine. SAXENA et al [13] explored the use of wet ethanol as a fuel for HCCI engines using exhaust heat recovery to provide the high input energy required for igniting wet ethanol. The results suggested that the best operating conditions for the HCCI engine occur with high intake pressures, high equivalence ratios, and highly delayed combustion timings.
Although several researches have been performed in modeling the HCCI engines, there are still tough challenges in the successful operation of HCCI engines, such as controlling the combustion phasing, extending the operating range, and high unburned hydrocarbon and CO emissions. Several control techniques have been proposed by researchers to control the combustion phase of HCCI engines such as inlet air heating, supercharging, equivalence ratio, exhaust gas recirculation (EGR), fuel reforming and compression ratio. Accordingly, massive research throughout the world has led to great progress in the control of HCCI combustion.
Associating with these controlling parameters, MARTINEZ-FRIAS et al [14] elaborated the ignition timing control system that involved varying the intake temperature of an HCCI engine. Their control system consisted of an exhaust-fresh charge heat exchanger, a supercharger, an EGR addition valve, and an intercooler. SU et al [15] presented a computational model to simulate a HCCI engine with direct injection (DI) during gas exchange. The new model is applied to estimate the influence of engine operating parameters such as the relative air–fuel ratio and early direct injection timing on HCCI combustion and emissions. KONG [16] studied the combustion characteristics of natural gas and dimethyl ether (DME) mixture in a HCCI engine. Combustion, nitrogen oxides emissions and effects of fuel compositions on engine operating limits were well predicted by the present model.
MACHRAFI and CAVADIASA [17] investigated the influence of inlet temperature, the equivalence ratio and the compression ratio on the pressure, the heat release and the ignition delays. The inlet temperature was changed from 25 to 70 °C and the equivalence ratio from 0.18 to 0.41, while the compression ratio varied from 6 to 13.5. KOMNINOS [18] presented a multi-zone model for HCCI combustion using a reduced set of chemical reactions coupled with a chemical kinetics solver. In this research, the equivalence ratio and the boost pressure were varied while engine speed was maintained constant. NOBAKHT et al [19] conducted a parametric study on natural gas HCCI combustion in order to identify the effect of inlet temperature and pressure, compression ratio, equivalence ratio and engine speed on combustion and engine performance parameters. Results revealed that among the considered parameters, the equivalence ratio and inlet pressure are the most valuable parameters which can improve the combustion and performance characteristics of the HCCI engine. FATHI et al [20] used a single cylinder engine to be operated in HCCI combustion mode fueled by natural gas and n-heptane. The main goal of the experiments was to investigate the possibility of controlling combustion phasing and combustion duration using various EGR fractions. Under examined condition, EGR addition improved fuel economy, reduced NOx emissions and increased HC and CO emissions.
In the recent study, RAHBARI et al [21–23] studied the effect of EGR, fuel reforming and compression ratio on HCCI combustion characteristics such as ignition timing, burn duration, temperature, pressure and NOx emission containing ethanol as fuel. ZHENG and CATON [24] used a single zone thermodynamic model with detailed chemical kinetics to determine the effect of operating parameters on nitrogen oxides emissions. Several sets of parametric studies were completed to investigate the effect of engine load, engine speed, equivalence ratio, EGR level, temperature, and fuel compositions on nitrogen oxides emissions. GHORBANPOUR and RASEKHI [25] simulated the HCCI engine by the CFD model and studied the influences of various important parameters such as injection timing, air–fuel equivalence ratio, injection rate shape and EGR on HCCI combustion.
In this work, two models are considered in order to predict the performance of HCCI engines fueled with ethanol. First, a thermodynamic model based on the two-step reaction mechanisms is improved in modeling the combustion process for which Arrhenius reaction rates are used. In the other model, a special attention has been paid on the effects of inlet temperature and equivalence ratio on ignition timing, burn duration, in- cylinder temperature and pressure of ethanol using chemical kinetic mechanism model. Also some efforts have been made to determine the dominant parameters able to control HCCI combustion process.
2 Two-step reaction mechanisms
In this approach, an instantaneous combustion at constant cylinder volume
is assumed and the rate of change of concentration for each species is given by the following equation:
(1)
In this case, the reaction rate expressions depend directly on both species concentration and cylinder temperature using Arrhenius-type relations. WESTBROOK and DRYER [26–27] derived two-step reaction mechanisms with hydrocarbon fuels. They concluded that this mechanism may successfully be used to predict combustion behaviour of hydrocarbon fuels. They also presented the following two-step mechanism reactions for ethanol as fuel:
(2)
(3)
The reaction rates for C2H6O and CO oxidations are given in the form of Eqs. (4) and (5) as introduced by [9]

(4)

(5)
The reaction rates of other species can be determined from the following relations:
(6)
(7)
(8)
(9)
3 Chemical kinetic mechanism model
The general equations governing conservations of mass, species and energy will be developed for a variable volume reactor, as shown in Fig. 1. It is assumed that the working fluid behaves as an ideal gas.
3.1 Conservation of mass
The rate of change of mass within any control volume is the net flux of mass across the system boundaries:
(10)
3.2 Conservation of species
Equations tracking the evolution of species within the combustion chamber will be developed on a mass basis according to Unlike CI and SI engines, the combustion process in a homogenous charge compression ignition engine is not controlled by mixing or entrainment. As a result, the rate of heat release is solely depended on the chemical kinetic reaction rates. To model the combustion process of HCCI engine, it is necessary to describe the heat release by a suitable chemical kinetic mechanism [28–29], and a well-matched, stiff chemical kinetic solver. The model includes the effects of heat transfer, for different engine configurations. The rates of creation/destruction of chemical species are modelled using mass-action kinetics and the specific reaction rate constants strongly depend on the temperature. An elementary reaction that involves k chemical species in i reactions can be represented in the form of
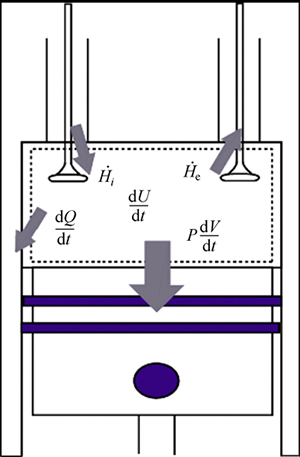
Fig. 1 Schematic view of control volume interaction with surrounding
(11)
(12)
(13)
(14)
(15)
where υki, χk,
qi, kf,i and kr,i are the stoichiometric coefficients, the chemical symbol for the kth species, the production rate of the kth species, the rate of progress variable for the ith reaction, the forward and reverse rate constants of the ith reaction, respectively.
The forward and reverse rate constants of the ith reaction rate could be expressed as
(16)
(17)
(18)
(19)
where Ai, βi, Ei and Kp,i are the pre-exponential factor, the temperature exponent, the activation energy and the equilibrium constants, respectively. The mass conservation equation for each species is also employed:
(20)
3.3 Thermodynamic property treatment
The working fluid composition in the cylinder is constantly changed. Once at any time the composition is determined, mixture properties can be calculated. This method simply considers thermodynamic properties of the mixture containing residual gas, exhaust gas recirculation, unburned gaseous fuel and air. For thermodynamic properties such as constant pressure specific heat, enthalpy and entropy, the NASA curve fits in the following forms are used:
(21)
(22)
(23)
3.4 Conservation of energy
The generalized energy equation for a thermodynamic control volume may be written as
(24)
The ideal gas law can be written as
(25)
These equations can be solved using Dvode subroutine and the ignition timing, burn duration, in-cylinder temperature and pressure can be extracted.
4 Results and verification
For the purpose of finding the accuracy of the present model, the predicted values of in-cylinder pressure for ethanol are compared with the experimental results obtained in Ref. [30]. The specifications of the engine used in the experiments as well as test conditions are presented in Table 1.
Table 1 Engine specifications [30]
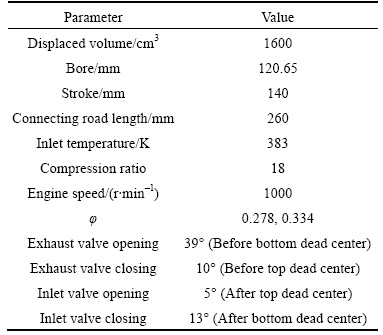
4.1 Cylinder pressure
Variations of pressure with crank angle are shown in Figs. 2 and 3 for φ=0.278 and φ=0.334, respectively. As seen, the maximum predicted pressures for thermodynamic model are about 71.3×105 Pa and 79.79×105 Pa and for chemical kinetic model they are about 71.48×105 Pa and 78.123×105 Pa, fairly comparable with corresponding experimental values of 72×105 Pa and 78.7×105 Pa. Theoretical results show that the combustion process starts at 12° (BTDC) and ends at 0.5° (ATDC) with a duration of 12.5° on crank angle for φ=0.278. The corresponding values for φ=0.334 are 11° (BTDC) and 0.3° (ATDC) and 11.3° on crank angle, respectively. Combustion durations of 12.5° and 11.3° obtained from theoretical model agree with experimental combustion durations of about 11° and 10°, respectively, concluded in Ref. [30].
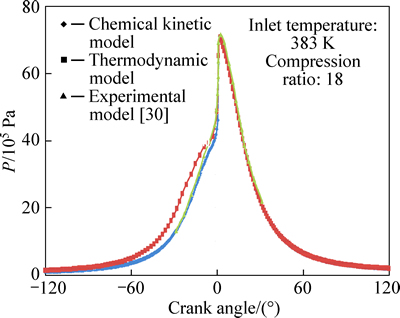
Fig. 2 Variation of predicted and experimental values of pressure with crank angle for φ=0.278
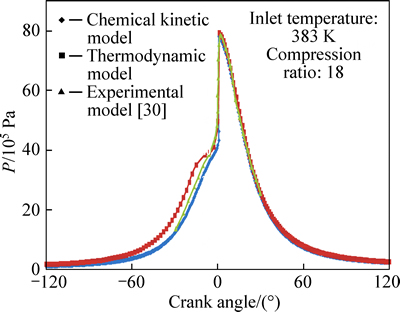
Fig. 3 Variation of predicted and experimental values of pressure with crank angle for φ=0.334
4.2 Cylinder temperature
Variations of temperature with crank angle for equivalence ratios of 0.278 and 0.334 are shown in Figs. 4 and 5, respectively. The maximum combustion temperatures of 1745 K and 1880 K predicted by the thermodynamic and chemical kinetic models for φ=0.278 and temperatures of 1920 K and 2020 K for φ=0.334 show that increase in φ will result in higher combustion temperatures, which is compatible with the fact that the richer mixtures provide higher combustion temperatures.
5 HCCI control techniques
In order to investigate the effects of important parameters on HCCI engine performance, predictedvalues of in-cylinder pressure and temperature as well as ignition timing and burn duration at different running conditions are obtained and results are compared with corresponding experimental data for the same engine specifications published in Ref. [31] and presented in Table 2.
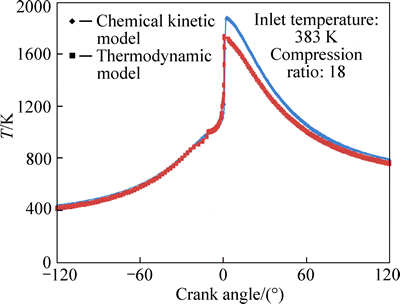
Fig. 4 Variation of predicted values of temperature with crank angle for φ=0.278
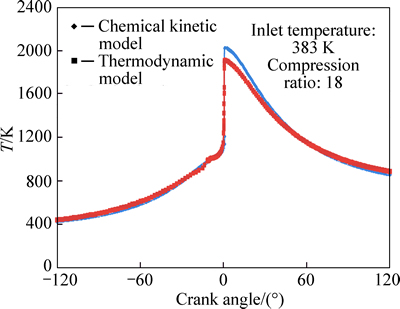
Fig. 5 Variation of predicted values of temperature with crank angle for φ=0.334
Table 2 Engine specifications [31]
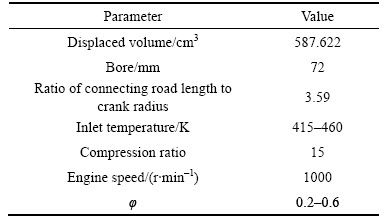
5.1 Effect of inlet temperature
5.1.1 Ignition timing and burn duration
Increasing the initial temperature (Tin) of the charge advances ignition timing, as shown in Fig. 6. Below Tin=421 K, no ignition can be achieved. Since HCCI combustion is controlled by chemical kinetics, the time at which the mixture overcomes the threshold energy is crucial to the combustion. The initial temperature of the engine charge is, in fact, the most important factor that affects ignition timing and the entire burning process. This is particularly important under conditions near the ignition limit of 421 K where a 5 K change in initial charge temperature advances the ignition by 3° on crank angle (See Fig. 6). Figure 7 shows variation of burn duration with initial temperature. It can be seen that the burn duration decreases rapidly with increase in initial temperature. This is due to the fact that the burn duration is related to the rate of reaction, which increases quicklywith increase in maximum cylinder temperature caused by higher initial charge temperature.
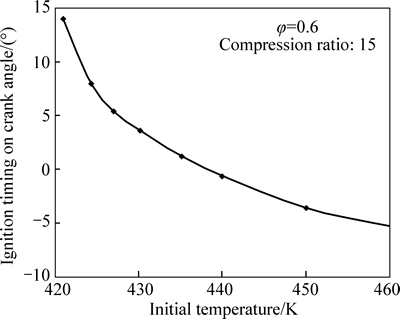
Fig. 6 Variation of ignition timing with initial charge temperature
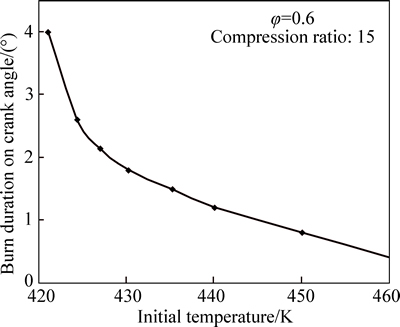
Fig. 7 Variation of burn duration with initial charge temperature
5.1.2 In-cylinder temperature and pressure
Figures 8 and 9 show that increasing the initial charge temperature increases the maximum cylinder temperature. As in the case of ignition timing, the changes are more dramatic when close to the combustion limit. The peak pressure continues to increase as peak pressure location moves towards top dead center (TDC)and the maximum peak pressure is achieved at TDC after which the peak pressure gradually decreases. It should be reminded that in-cylinder temperature and pressure are related through ideal gas law and both are mainly affected by heat release rate and chemical kinetics.
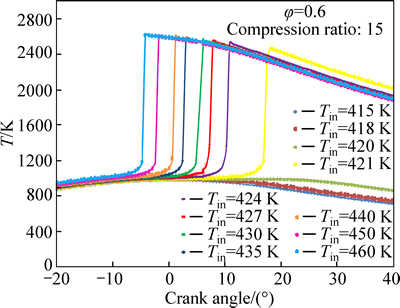
Fig. 8 Effect of initial temperature on temperature profile
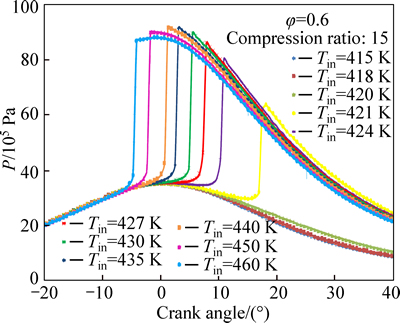
Fig. 9 Effect of initial temperature on pressure profile
5.2 Effect of equivalence ratio
5.2.1 Ignition timing and burn duration
Variations of ignition timing with initial charge temperature are shown in Fig. 10 for equivalence ratios of 0.2 and 0.6. As seen, a minimum initial temperature is required in order to initiate the combustion process. This minimum temperature is lower for leaner mixtures so that an initial temperature as low as 415 K is enough to ignite a mixture with equivalence ratio of 0.2. In fact, a minimum concentration of radicals is needed to raise the compression process temperature to a level high enough to ignite the mixture. These radicals are produced by chain reactions and molecular dissociations which have higher rates in leaner mixtures, and as a result, a more advanced initiation of combustion will be achieved.
Figure 11 shows variation of burn duration with equivalence ratio. As observed, the burn duration decreases with increase in equivalence ratio as a result of the higher reaction rate produced by the higher equivalence ratio.
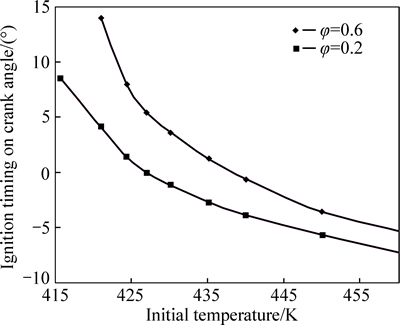
Fig. 10 Variation of ignition timing with initial charge temperature for equivalence ratios of 0.2 and 0.6
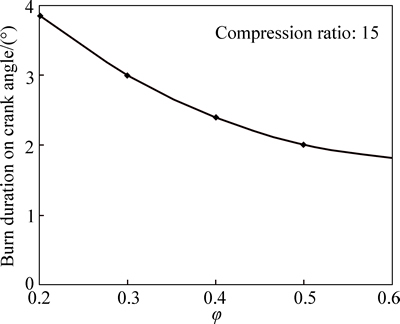
Fig. 11 Variation of burn duration with equivalence ratio
5.2.2 In-cylinder temperature and pressure
Variations of in-cylinder temperature with crank angle for different equivalence ratios are shown in Fig. 12. The less chemical energy associated with leaner mixtures is the main reason for decreasing the combustion temperature. As seen in Fig. 13, the peak pressure decreases with decrease in equivalence ratio especially at very lean mixtures. Although mixtures with very low equivalence ratios (φ=0.2) can be ignited at lower temperatures, the combustion efficiency is very low at the points near the ignition limit.
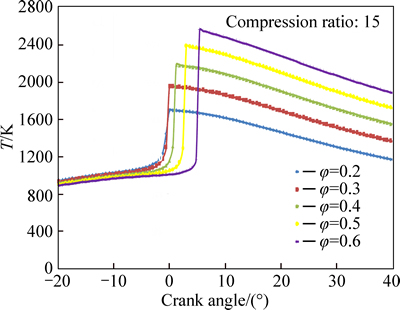
Fig. 12 Effect of equivalence ratio on temperature profile
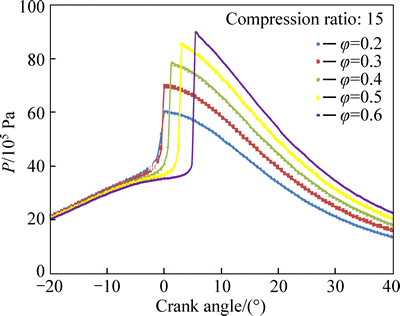
Fig. 13 Effect of equivalence ratio on pressure profile
The initiation of combustion is much more advanced and combustion duration is much longer with leaner mixtures. These two key parameters affect the in-cylinder temperature and pressure profiles and dictate the positions of maximum combustion temperature and pressure. As seen from Figs. 12 and 13, the maximum temperature and pressure are associated with richer mixtures, however, for leaner mixtures, the points of peak pressure and temperature are closer to TDC.
6 Conclusions
1) It is shown that the predicted values are in good agreement with corresponding experimental data.
2) The chemical kinetic model is more accurate in predicting the engine performance compared to the thermodynamic model.
3) After validating data, the impacts of inlet temperature and equivalence ratio on the combustion characteristics of HCCI engines fuelled with ethanol are obtained.
4) It is declared that the intake charge heating is the most effective combustion controller and there is a minimum intake temperature for each operating condition in order to achieve the stable combustion.
5) Increasing the initial temperature advances the ignition timing, decreases the burn duration and increases the peak temperature and pressure.
6) It is observed that the minimum initial temperature is required to initiate the combustion process for each equivalence ratio and this minimum temperature is lower for leaner mixtures.
7) The burn duration declines with augmentation in equivalence ratio.
8) Consequently, the maximum temperature and pressure are associated with richer mixtures, however, for leaner mixtures, the location of peak pressure and temperature are closer to TDC.
References
[1] MAURYA R, AGARWAL A. Experimental investigations of performance, combustion and emission characteristics of ethanol and methanol fueled HCCI engine [J]. Fuel Processing Technology, 2014, 126: 30–48.
[2] YAO M, ZHENG Z, LIU H. Progress and recent trends in homogeneous charge compression ignition engines [J]. Progress in Energy and Combustion Science, 2009, 35:398–437.
[3] CHRISTENSEN M, JOHANSSON B, EINEWALL P. Homogeneous charge compression ignition (HCCI) using iso-octane, ethanol and natural gas, a comparison with spark ignition operation [R]. SAE 972824, 1997.
[4] CHRISTENSEN M, HULTQVIST A, JOHANSSON B. Demonstrating the multi fuel capability of a homogenous charge compression ignition engine with variable compression ratio [R]. SAE 1999–01–3679, 1999.
[5] MACK J H, FLOWERS D L, BUCHHOLZ B A, DIBBLE R W. Investigation of HCCI combustion of diethyl ether and ethanol mixtures using carbon 14 tracing and numerical simulations [J]. Proceedings of the Combustion Institute, 2005, 30: 2693–2700.
[6] YAP D, MEGARITIS A. Applying forced induction to bioethanol HCCI operation with residual gas trapping [J]. Energy Fuels, 2005, 19: 1812–1821.
[7] YAP D, MEGARITIS A, WYSZYNSKI M L. An experimental study of bioethanol HCCI [J]. Combustion Science and Technology, 2005, 177: 2039–2068.
[8] MEGARITIS A, YAP D, WYSZYNSKI M L. Effect of water blending on bioethanol HCCI combustion with forced induction and residual gas trapping [J]. Energy, 2007, 32: 2396–2400.
[9] MACK J, ACEVES S M, DIBBLE R W. Demonstrating direct use of wetethanolin a homogeneous charge compression ignition (HCCI) engine [J]. Energy, 2009, 34:782–787.
[10] KOMNINOS N P, RAKOPOULOS C D. Comparison of a detailed and a reduced ethanol oxidation mechanism in HCCI combustion using a multi-zone model [J]. The Open Renewable Energy Journal, 2011, 4: 47–59.
[11] KHALIQ A, TRIVEDI S K, DINCER I. Investigation of a wet ethanol operated HCCI engine based on first and second law analyses [J]. Applied Thermal Engineering, 2011, 31: 1621–1629.
[12] VIGGIANO A, MAGI V. A comprehensive investigation on the emissions ofethanol HCCI engines [J]. Applied Energy, 2012, 93:277–287.
[13] SAXENA S, SCHNEIDER S, ACEVES S, DIBBLE R. WetethanolinHCCI engineswith exhaust heat recovery to improve the energy balance of ethanol fuels [J]. Applied Energy, 2012, 98:448–457.
[14] MARTINEZ-FRIAS J, ACEVES S M, FLOWERS D, SMITH J R, DIBBLE R. Exhaust energy recovery for control of a homogeneous charge compression ignition engine [J]. ASME, 2000, 40: 349–356.
[15] SU H, VIKHANSKY A, MOSBACH S, KRAFT M, BHAVE A, KIM K, KOBAYASHI T, MAUSS F. A computationalstudyof anHCCI enginewith direct injection during gas exchange [J]. Combustion and Flame, 2006, 147:118–132.
[16] KONG S C. Astudyof natural gas/DME combustion inHCCI enginesusing CFD with detailed chemical kinetics [J]. Fuel, 2007, 86:1483–1489.
[17] MACHRAFI H, CAVADIASA S. An experimental and numerical analysis of the influence of the inlet temperature, equivalence ratio and compression ratio on the HCCI auto-ignition process of primary reference fuels in an engine [J]. Fuel Processing Technology, 2008, 89: 1218–1226.
[18] KOMNINOS N P. ModelingHCCIcombustion: Modification of a multi-zone model and comparison to experimental results at varying boost pressure [J]. Applied Energy, 2009, 86: 2141–2151.
[19] NOBAKHT A Y, SARAY R K, RAHIMI A. A parametric study on natural gas fueled HCCI combustion engine using a multi-zone combustion model [J]. Fuel, 2011, 90:1508–1514.
[20] FATHI M, SARAY R K, CHECKEL M D. The influence of exhaust gas recirculation (EGR) on combustion and emissions of n-heptane/natural gas fueled homogeneous charge compression ignition (HCCI) engines [J]. Applied Energy, 2011, 88:4719–4724.
[21] RAHBARI A. The effect of EGR on HCCI engines using ethanol as fuel [R]. SAE 2008–01–2409, 2008.
[22] RAHBARI A, BARARI B, SHIRAZI A. Computational investigation of HCCI engine performance with fuel reforming effect on lean ethanol [R]. ASME ICES2009-76142, 2009.
[23] RAHBARI A, AFSARI A, BARARI B, ROSTAMSOWLAT I. Theoretical investigation over the effects of EGR, fuel reforming and compression ratio on the HCCI engine operation fuelled with ethanol [J]. Global Journal of Researches in Engineering, 2012, 12: 1–13.
[24] ZHENG J, CATON J A. Effects of operating parameters on nitrogen oxides emissions for a natural gas fueled homogeneous charged compression ignitionengine (HCCI): Results from a thermodynamic model with detailed chemistry [J]. Applied Energy, 2012, 92:386–394.
[25] GHORBANPOUR M, RASEKHI R. A parametric investigation ofHCCIcombustion to reduce emissions and improve efficiency using a CFD model approach [J]. Fuel, 2013, 106:157–165.
[26] WESTBROOK C K, DRYER F L. Simplified reaction mechanisms for the oxidation of hydrocarbon fuels in flames [J]. Combustion Science and Technology, 1981, 27: 31–43.
[27] WESTBROOK C K. Chemical kinetics of hydrocarbon ignition in practical combustion systems [J]. Proceedings of the Combustion Institute, 2000, 28: 1563–1577.
[28] LI J, KAZAKOV A, CHAOS M, DRYER F L. Chemical kinetics of ethanol oxidation [C]// 5th US Combustion Meeting. 2007.
[29] MARINOV M. A detailed chemical kinetic model for high temperature ethanol oxidation [J]. International Journal of Chemical Kinetics, 1999, 31:183–220.
[30] CHRISTENSEN M, JOHANSSON B. Influence of mixture quality on homogenous charge compression ignition [R]. SAE 982454, 1998.
[31] GNANAM G, JOHANSON M, SOBIESIAK A, READER G. HCCI combustion with internal fuel reforming, varied levels of EGR and charge preheat–A computational study [R]. SAE 2005–01–0140, 2005.
(Edited by YANG Bing)
Received date: 2015-03-03; Accepted date: 2015-06-19
Corresponding author: Alireza Rahbari; Tel: +98–912–1344361; Fax: +98–21–77240488; E-mail: ar.rahbari@gmail.com