
Electrochemical performance of nickel hydroxide doped with multi-wall carbon nanotubes
LI Yan-wei(李延伟)1, 2, YIN Zhou-lan(尹周澜)1, YAO Jin-huan(姚金环)2,
ZHAO Wei-min(赵卫民)2, LIU Chang-jiu(刘长久)2, ZHONG Sheng-kui(钟胜奎)1, 2
1. School of Chemistry and Chemical Engineering, Central South University, Changsha 410083, China;
2. College of Chemistry and Bioengineering, Guilin University of Technology, Guilin 541004, China
Received 6 July 2009; accepted 30 December 2009
_____________________________________________________________________________________________________
Abstract: Nickel hydroxide doped with multi-wall carbon nanotubes (MCNTs) was synthesized by chemical coprecipitation method. The MCNTs doped nickel hydroxide was used as the electrochemical active material in the positive electrodes of rechargeable alkaline batteries. The powder X-ray diffraction (XRD) analysis shows that the addition of MCNTs induces more structural defect within the crystal lattice of the nickel hydroxide. The cyclic voltammetry (CV) and electrochemical impedance spectroscopy (EIS) tests demonstrate the better reaction reversibility and lower electrochemical impedance of MCNTs doped nickel hydroxide as compared with the pure nickel hydroxide. The charge/discharge tests show that MCNTs addition can improve the specific discharge capacity and increase the discharge voltage of the nickel hydroxide electrode.
Key words: nickel hydroxide; carbon nanotube; Ni/MH batteries; specific capacity; electrochemical impedance spectroscopy
_____________________________________________________________________________________________________
1 Introduction
Nickel hydroxide is widely used as the positive electrode material in rechargeable nickel-based batteries, which plays an important role in the field of electric energy storage devices. Besides the very diverse applications including portable electronics, power tools, remote power systems, satellites, and personal transportations, the nickel-based battery system appears to be one of the technologies of choice for the emerging electric vehicles (EVs) and hybrid electric vehicles (HEVs)[1]. Nickel/metal hydride (Ni/MH) batteries are considered to be one of the most promising choices for EVs and HEVs applications because of their better combination of output power, capacity, life, reliability, and cost[2]. In such situations, the high-proton diffusion coefficients and high-electronic conductivity are undoubtedly the key factors for its use. Nickel hydroxide (β-nickel hydroxide), the active material of positive electrode of nickel-based batteries, is a kind of p-type semiconductor and its poor electronic conductivity handicaps the high-rate dischargeability of Ni/MH batteries. To improve the electronic conductivity of the nickel hydroxide, many attempts have been made in recent years, among which the most commonly used approach is to add cobalt additive, such as CoO and Co(OH)2, directly to the positive electrode so as to form a CoOOH conductive film on the surface of nickel hydroxide particles[3-5]. It should be noted that this physical mixture of nickel hydroxide and additives is not effective to reduce the inner resistance of the particles.
Carbon nanotubes (CNTs) have high electrical conductivity, excellent mechanical properties, and bending strength[6]. Because of these excellent properties, CNTs have attracted much attention as a promising material for different energy storage/ conversion systems, such as electrode materials for supercapacitors[7], hydrogen storage materials for Ni/MH batteries and fuel cells[8], and electrode materials for lithium batteries[9]. L? et al[10] firstly attempted to use CNTs as an additive in the nickel electrode, and investigated the influence of CNTs on the high-rate discharge properties of type-AA Ni/MH batteries. They found that the addition of CNTs may improve the performance of batteries at the high discharge rate. SONG et al[11] added CNTs to spherical β-nickel hydroxide powder as active material to prepare the pasted electrodes. They found that the CNTs addition can increase the material utilization and specific discharge capacity of nickel hydroxide. Nevertheless, in the above studies the utilization way is still a physical mixture of active material and CNTs. Therefore, the utilization efficiency of CNTs is relatively low because it is difficult to achieve sufficient contact between the conductor and active material. In the present study, we synthesized nickel hydroxide/CNTs composites, in which the CNTs mixed homogenously with nickel hydroxide, by chemical coprecipitation method. The microstructure and electrochemical performance of the CNTs doped nickel hydroxide were investigated by X-ray diffractometry (XRD), cyclic voltammetry (CV), electrochemical impedance spectroscopy (EIS), and charge/discharge tests.
2 Experimental
2.1 Synthesis and characterization of CNTs doped nickel hydroxide
All of the reagents used except CNTs were of analytical purity and used without further purification. CNTs used in this study were obtained from Shenzhen Nanotech Port Co. Ltd., China (Multi-wall carbon nanotubes, purity≥95%, ash≤0.2%, mass fraction). The MCNTs were purified by immersing in a concentrated nitric acid for 48 h, and then washed with de-ionized water, dried to constant mass at 60 ℃ in a vacuum drier. The nickel hydroxide/CNTs composite was synthesized by chemical coprecipitation method. NaOH aqueous solution (2 mol/L) and NiSO4 aqueous solution (1 mol/L) were added dropwise into an ethanol solution with MCNTs in the given ratio (mass ratio of Ni(OH)2 to MCNTs is 100:1) under constant stirring. To improve the dispersion of MCNTs, the ethanol solution was treated by ultrasonic for 30 min before adding. The precipitation reaction was carried out at 60 ℃ and constant stirring for 6 h. Then the suspension obtained was kept in the mother solution for 10 h and allowed to settle. The precipitate was then filtrated, washed with distilled water, and dried at 60 ℃ for 24 h. For comparison, pure nickel hydroxide was also synthesized by the same chemical coprecipitation process without the addition of MCNTs. The same reagents as above were used.
The crystal structure of the prepared powders was characterized by X-ray diffraction (XRD) analysis using X’ Pert Pro diffractometer, with a Cu Kα radiation source (λ=0.145 81 nm, V=40.0 kV, I=100 mA).
2.2 Preparation of nickel electrode and electro- chemical tests
The prepared nickel hydroxide/MCNTs composite or pure nickel hydroxide as active material was mixed with 10% (mass fraction) nickel powder. Proper amount of binder (6% PTFE, mass fraction) and distilled water were then added into the mixture to obtain a homogeneous slurry with adequate rheological properties. The slurry was filled into a foam nickel sheet (1 cm × 1.5 cm) and then dried at 80 ℃ for 1 h. Subsequently, the pasted electrodes were pressed at 8 MPa for 3 min to assure good electrical contact between the foam nickel and active material.
Charge/discharge studies were conducted in a test cell, including the prepared nickel electrodes as cathode and a hydrogen-storage alloy electrode as the anode. Polypropylene was used as the separator between the cathode and anode. The electrolyte was 6 mol/L KOH + 18 g/L LiOH solution. Charge/discharge measurements were conducted using a Land CT2001 C battery performance-testing instrument at room temperature. The charge was done at 0.2C for 6 h with a charge limiting voltage of 1.5 V. The discharge was done at 0.2C and the cut-off voltage was chosen to be 1.0 V. Cyclic voltammetry (CV) and electrochemical impedance spectra (EIS) measurements were performed on auto-lab electrochemistry workstation in a three-electrode system with a Hg/HgO as reference electrode and a hydrogen- storage alloy counter electrode. The electrolyte was 6 mol/L KOH + 18 g/L LiOH solution. In CV measurement, the scan potential ranged from 0.1 to 0.7 V and the scanning rate was 1 mV/s. In EIS measurement, the impedance spectra were recorded at 5 mV amplitude of perturbation with a sweep frequency range from 10 kHz to 10 mHz. All the measurements were done at room temperature.
3 Results and discussion
3.1 Microstructure of prepared nickel hydroxide
Fig.1 shows the XRD patterns of the prepared nickel hydroxide samples. It can be seen that the diffraction peaks of both samples are indexed as the hexagonal phase of typical β-nickel hydroxide (JCPDS-14-0117). Compared with the pure nickel hydroxide, the diffraction peaks of the MCNTs doped nickel hydroxide are noticeably broadened. This broadening of the diffraction peaks can be attributed to the increased crystal defects because of the existence of stack faults and the presence of other polymorphic modification as interstratified phases[12-13]. It has been reported that the crystalline state of nickel hydroxide has a significant influence on its electrochemical performance[14-16]. These structural defects and disorder within the crystal lattice of the MCNTs doped nickel hydroxide are inferred to improve the electrochemical performance, which can be further proved by the following CV, EIS, and charge/discharge tests.
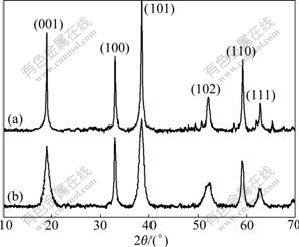
Fig.1 XRD patterns of pure nickel hydroxide (a) and MCNTs doped nickel hydroxide (b)
3.2 CV and EIS measurements of nickel electrodes
The CV curves of nickel electrodes are shown in Fig.2. For both electrodes, one anodic nickel hydroxide oxidation peak, appearing at 450-500 mV, is recorded before oxygen evolution. Similarly, one cathodic oxyhydroxide reduction peak at 250-290 mV is observed on the reverse sweep. Generally, the potential difference (?E) between the anodic and cathodic peak potentials can be used to characterize the reversibility of the redox reaction[17]. In comparison with that of the pure nickel hydroxide electrode, the anodic peak potential of MCNTs doped nickel hydroxide electrode is obviously shifted to a more negative value, while the cathodic potential is shifted to a more positive value. The ?E values for the MCNTs doped nickel hydroxide electrode and pure nickel hydroxide electrode are 163 mV and 234 mV, respectively, suggesting that the MCNTs doped nickel hydroxide has better reversibility of the electrode reaction.
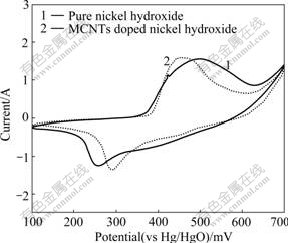
Fig.2 Cyclic voltammograms of pure nickel hydroxide and MCNTs doped nickel hydroxide with scanning rate of 1 mV/s-1
Fig.3 presents the electrochemical impedance spectra (EIS) of the pure nickel hydroxide electrode and MCNTs doped nickel hydroxide electrode at the steady state after being activated by cyclic voltammetric test. The impedance spectra of these two electrodes display a depressed semicircle from the charge transfer resistance in the high-frequency region, and a slope related to the Warburg impedance appearing in the low-frequency region[18-19]. It is observed from Fig.3 that the pure nickel hydroxide electrode exhibits a much larger capacitive semicircle than the MCNTs doped nickel hydroxide electrode. This implies that the addition of MCNTs can dramatically decrease the charge transfer resistance and the electrochemical reaction within the MCNTs doped nickel hydroxide proceeds more easily than that within the pure nickel hydroxide.
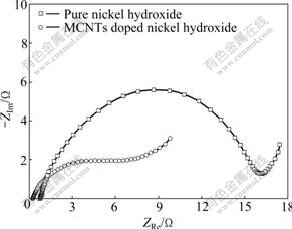
Fig.3 Electrochemical impedance spectra of pure nickel hydroxide electrode and MCNTs doped nickel hydroxide electrode
Just as previously discussed, the MCNTs doped nickel hydroxide possesses more structural defects, which can provide higher proton diffusion coefficients and ionic conductivity. On the other hand, the MCNTs distributed in nickel hydroxide reduce the inner resistance of nickel hydroxide particles due to its high accessible surface and good electrical conductivity. All these factors facilitate the intercalation/de-intercalation of protons or the rapid movement of both electrons and protons in the electrode. Accordingly, the smaller electrochemical reaction impedance and better reaction reversibility can be obtained for MCNTs doped nickel hydroxide electrode.
3.3 Charge/discharge test of nickel electrode
Charge/discharge tests of the simulated batteries with pure nickel hydroxide electrode and MCNTs doped nickel hydroxide electrode were carried out at 0.2C with a charging limiting voltage of 1.5 V and a discharge cut-off voltage of 1.0 V. Fig.4 shows the typical discharge curves of the two nickel electrodes. It can be seen from Fig.4 that the specific discharge capacity of the MCNTs doped nickel hydroxide electrode (267 mA?h/g) is larger than that of the pure nickel hydroxide electrode (237 mA?h/g). Moreover, the discharge plateau of the MCNTs doped nickel hydroxide electrode is also higher than that of the pure nickel hydroxide electrode, which indicates that the MCNTs doped nickel hydroxide has smaller polarization during the discharge process.
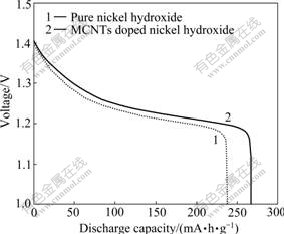
Fig.4 Discharge curves of pure nickel hydroxide and MCNTs doped nickel hydroxide at 0.2C rate
According to the discharge kinetics proposed by ZIMMERMAN and EFFA[20], the kinetics of nickel electrode is controlled by solid-state proton diffusion under the normal high rate discharge conditions. As the nickel electrode is discharged, the conductivity of the active material decreases until eventually mixed kinetics of both proton diffusion and charge transfer is observed. Further discharge results in the formation of a semiconductor layer at the metal-active material interface that is depleted in charge carriers and has a relatively high electronic resistance. A conducting network with stable electrochemical properties is important for providing enough current collectors in the positive electrode, which can decrease the charge transfer resistance. Due to the excellent electronic conductivity, high accessible surface area, and unique mechanical properties, the addition of MCNTs increases the ionic and electronic conductivity within nickel hydroxide, resulting in a larger discharge capacity and higher utilization of nickel hydroxide active material.
4 Conclusions
1) The addition of MCNTs in nickel hydroxide may result in more structural defects within the crystal lattice of nickel hydroxide.
2) The MCNTs doped nickel hydroxide has better reaction reversibility and lower electron transfer resistance as compared with that of pure nickel hydroxide.
3) The discharge capacity and discharge voltage of the MCNTs doped nickel hydroxide are higher than those of the pure nickel hydroxide.
References
[1] GIFFORD P, ADAMS J, CORRIGAN D, VENKATESAN S. Development of advanced nickel/metal hydride batteries for electric and hybrid vehicles [J]. J Power Source, 1999, 80: 157-163.
[2] CHAN C C. The state of the art of electric and hybrid vehicles [J]. Proc IEEE, 2002, 90: 247-275.
[3] WATANABE K, KOSEKI M, KUMAGAI N. Effect of cobalt addition to nickel hydroxide as a positive material for rechargeable alkaline batteries [J]. J Power Source, 1996, 58: 23-28.
[4] WANG X, YAN J, YUAN T, ZHOU Z, SONG D, ZHANG Y, ZHU L. Surface modification and electrochemical studies of spherical nickel hydroxide [J]. J Power Source, 1998, 72: 221-225.
[5] NATHIR BEGUM S, MURALIDHARAN V S, AHMED BASH C. The influences of some additives on electrochemical behaviour of nickel electrodes [J]. Int J Hydrogen Energy, 2009, 34: 1548-1555.
[6] BAUGHMAN R H, ZAKHIDOV A A, de HEER W A. Carbon nanotubes-the route toward applications [J]. Science, 2002, 297: 787-792.
[7] WANG X F, RUAN D B, YOU Z. Application of spherical Ni(OH)2/CNTs composite electrode in asymmetric supercapacitor [J]. Trans Nonferrous Met Soc China, 2006, 16: 1129-1134.
[8] SCHLAPBACH L, ZUTTEL A. Hydrogen-storage materials for mobile applications [J]. Nature, 2001, 414: 353-358.
[9] SCOTT MORRIS R, DIXON B G, GENNETT T, RAFFAELLE R, HEBEN M J. High-energy, rechargeable Li-ion battery based on carbon nanotube technology [J]. J Power Source, 2004, 138: 277-280.
[10] L? J, TU J P, ZHANG W K, WU J B, WU H M, ZHANG B. Effects of carbon nanotubes on the high-rate discharge properties of nickel/metal hydride batteries [J]. J Power Source, 2004, 132: 282-287.
[11] SONG Q S, ARAVINDARAJ G K, SULTAN H, CHANB S L L. Performance improvement of pasted nickel electrodes with multi-wall carbon nanotubes for rechargeable nickel batteries [J]. Electrochimica Acta, 2007, 53: 1890-1896.
[12] TESSIER C, HAUMESSER PH, BERNARD P, DELMAS C. The structure of Ni(OH)2: From the ideal material to the electrochemically active one [J]. J Electrochem Soc, 1999, 146: 2059-2067.
[13] DEABATE S, FOURGEOT F, HENN F. X-ray diffraction and micro-Raman spectroscopy analysis of new nickel hydroxide obtained by electrodialysis [J]. J Power Source, 2000, 87: 125-136.
[14] BERNARD M C, CORTES R, KEDDAM M, TAKENOUTI H, BERNARD P, SENYARICH S. Structural defects and electrochemical reactivity of β-Ni(OH)2 [J]. J Power Source, 1996, 63: 247-254.
[15] DELMAS C, TESSIER C. Stacking faults in the structure of nickel hydroxide: A rationale of its high electrochemical activity [J]. J Mater Chem, 1997, (7): 1439-1443.
[16] RAMESH T N, VISHNU KAMATH P, SHIVAKUMARA C. Correlation of structural disorder with the reversible discharge capacity of nickel hydroxide electrode [J]. J Electrochem Soc, 2005, 152: A806-A810.
[17] VISHNU KAMATH P, SUBBANNA G N. Electroless nickel hydroxide: Synthesis and characterization [J]. J Appl Electrochem, 1992, 22: 478-482.
[18] ZHANG Y, ZHOU Z, YAN J. Electrochemical behaviour of Ni(OH)2 ultrafine powder [J]. J Power Source, 1998, 75: 283-287.
[19] WANG X Y, YAN J, YUAN H T, ZHANG Y S, SONG D Y. Impedance studies of nickel hydroxide microencapsulated by cobalt [J]. Inter J Hydrogen Energy, 1999, 24: 973-980.
[20] ZIMMERMAN A H, EFFA P K. Discharge kinetics of the nickel electrode [J]. J Electrochem Soc, 1984, 131: 709-713.
________________________
Foundation item: Project(20090450188) supported by China Postdoctoral Science Foundation; Project supported by Postdoctoral Science Foundation of Central South University, China; Project(0991247) supported by Natural Science Foundation of Guangxi Province, China
Corresponding author: LI Yan-wei; Tel: +86-773-5896446; E-mail: lywhit@glite.edu.cn
(Edited by LI Xiang-qun)