J. Cent. South Univ. Technol. (2010) 17: 1172-1176
DOI: 10.1007/s11771-010-0614-1
Structures and magnetic properties of nanocomposite CoFe2O4-BaTiO3 fibers by organic gel-thermal decomposition process
ZHOU Zhi(周智), SHEN Xiang-qian(沈湘黔), SONG Fu-zhan(宋福展), MIN Chun-ying(闵春英)
School of Materials Science and Engineering, Jiangsu University, Zhenjiang 212013, China
? Central South University Press and Springer-Verlag Berlin Heidelberg 2010
Abstract: The nanocomposite xCoFe2O4-(1-x)BaTiO3 (x=0.2, 0.3, 0.4, 0.5, molar fraction) fibers with fine diameters and high aspect ratios (length to diameter ratios) were prepared by the organic gel-thermal decomposition process from citric acid and metal salts. The structures and morphologies of gel precursors and fibers derived from thermal decomposition of the gel precursors were characterized by Fourier transform infrared spectroscopy, X-ray diffractometry and scanning electron microscopy. The magnetic properties of the nanocomposite fibers were measured by vibrating sample magnetometer. The nanocomposite fibers consisting of ferrite (CoFe2O4) and perovskite (BaTiO3) are formed at the calcination temperature of 900 ℃ for 2 h. The average grain sizes of CoFe2O4 and BaTiO3 in the nanocomposite fibers increase from 25 to 65 nm with the calcination temperature from 900 to 1 180 ℃. The single fiber constructed from these nanograins of CoFe2O4 and BaTiO3 has a necklace-like morphology. The saturation magnetization of the nanocomposite 0.4CoFe2O4-0.6BaTiO3 fibers increases with the increase of CoFe2O4 grain size, while the coercivity reaches a maximum value when the average grain size of CoFe2O4 is around the critical single-domain size of 45 nm obtained at 1 000 ℃. The saturation magnetization and remanence of the nanocomposite xCoFe2O4-(1-x)BaTiO3 (x=0.2, 0.3, 0.4, 0.5) fibers almost exhibit a linear relationship with the molar fraction of CoFe2O4 in the nanocomposites.
Key words: nanocomposite; CeFe2O4; BaTiO3; fiber; organic gel-thermal decomposition process; magnetic property
1 Introduction
Quasi-one-dimensional (Q1D) nanostructured materials have attracted much attention due to their chemical and physical properties and their potential as building blocks for advanced functional devices. In order to obtain Q1D materials, many different preparation techniques, such as arc discharge [1], laser ablation [2], template [3-4], metal organic chemical vapour deposition [5], electrospinning [6] and precursor thermal decomposition [7-8] were developed. The precursor thermal decomposition process due to simple operation and low cost was used to fabricate microscale fibers [9-10]. Among these microscale fibers, most of them were single phase materials [10]. Two and multiphase composite functional materials stimulated interests as these composite materials potentially combine the component characteristics to meet various requirements technologically. In particular, the multiferroic composite Q1D materials consisting of ferromagnetic and ferroelectric phases have been recently reported to show multifunctional features [11]. HUA et al [12] synthesized CoFe2O4/Pb (Zr0.52Ti0.48)O3(PZT) nanotubes by the sol- gel template process. The magnetic and electric performances are found to be similar to those of the single CoFe2O4 phase and PZT phase, respectively. XIE et al [13] prepared NiFe2O4/PZT nanofibers with diameters of 100-400 nm and grain sizes of about 30 nm by electrospinning. In this work, the nanocomposite CoFe2O4-BaTiO3 fibers were prepared by the organic gel-thermal decomposition process, and the structure and magnetic properties of these nanocomposite fibers were investigated.
2 Experimental
The nanocomposite xCoFe2O4-(1-x)BaTiO3 (x=0.2, 0.3, 0.4, 0.5, molar fraction) fibers were prepared by the organic gel-thermal decomposition process, and the process was described in detail in Refs.[9-10]. The starting reagents used were analytical grade Fe(NO3)3·
9H2O, Co(NO3)2·6H2O, BaCO3, Ti(OC4H9) and citric acid. The required metal salts and citric acid were dissolved in an aqueous and ethanol hybrid solution at pH 7.0 with a continuous magnetic stirring. The solution was magnetically stirred at room temperature for 20- 24 h and then surplus water in a vacuum rotary evaporator was removed at 60-80 ℃ until a viscous liquid was obtained. The gel fibers were drawn from the spinnable gels by the domestic machine and dried in a vacuum oven at 80 ℃ for about 24 h. The dried gel fibers were then put in an alumina crucible and subsequently calcined at appropriate temperatures for 2 h under an ambient atmosphere to form the nanocomposite fibers.
The structures, compositions and morphologies of the gel precursors and fibers were examined by Fourier transform infrared spectroscopy (FTIR) using a model of Nexu670 spectrometer, X-ray diffractometry (XRD) using a D/max2500PC diffractometer (RIGAKU), and scanning electron microscopy using a field emission scanning electron microscopy (FESEM, JSM-7001F). Magnetic measurements were carried out at room temperatures by a vibrating sample magnetometer (VSM).
3 Results and discussion
3.1 FTIR spectra of gel precursor and resultant fibers
Fig.1 shows the FTIR spectra of the gel precursor for 0.4CoFe2O4-0.6BaTiO3 fibers and the products derived from calcination of the precursor at different temperatures. From the FTIR spectrum for the gel precursor (Fig.1(a)), two bands at 1 624 and 1 383 cm-1 owing to the RCOO- symmetrical and asymmetrical stretching vibration are the characteristic peaks for the citrate. The bands at 1 040, 850, 825, 690 and 640 cm-1 can be assigned to the vibrations arising from C—OH, Ti—O, NO3-, Co—O and Fe—O bonds [14-16], respectively. This is indicative of the complex formation of metal ions and citric acid.
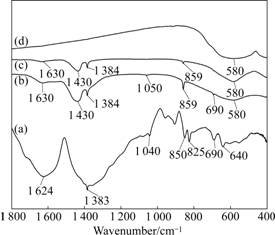
Fig.1 FTIR spectra of gel precursor and 0.4CoFe2O4-0.6BaTiO3 fibers calcined at different temperatures: (a) Gel precursor; (b) 300 ℃; (c) 800 ℃; (d) 900 ℃
For the sample obtained at 300 ℃, as shown in Fig.1(b), the characteristic absorption peak for the citrate disappears owing to the decomposition of citrate. Two bands at 1 430 and 859 cm-1 can be assigned to the characteristic absorption peaks for BaCO3, while the bands at 1 630, 1 384, 1 050, 690 and 580 cm-1 are corresponding to the characteristic vibration peaks of H2O, NO3-, Fe2O3, Co3O4 and TiO2 [17-19]. After calcination at 800 ℃ (Fig.1(c)), the peaks assigned to Fe2O3 and Co3O4 disappear and the characteristic peak occurs in the region of 550-600 cm-1 due to the stretching mode of Fe—O at tetrahedral sites in the spinel structure and the Ti—O stretching mode of BaTiO3 in the perovskite structure, which indicates that spinel structure CoFe2O4 and perovskite structure BaTiO3 begin to form at the calcination temperature of 800 ℃ [20-21]. As shown in Fig.1(d), when the calcination temperature increases up to 900 ℃, only the characteristic peak at around 580 cm-1 for CoFe2O4 and BaTiO3 is detected, implying the composite of CoFe2O4 and BaTiO3 formed.
3.2 Structural characterization of nanocomposite fibers
Fig.2 shows XRD patterns of the gel precursor and nanocomposite 0.4CoFe2O4-0.6BaTiO3 fibers obtained at various calcination temperatures for 2 h. It can be seen that the gel precursor (Fig.2(a)) is basically amorphous and does not contain crystalline inorganic matters. After calcination at 800 ℃ (Fig.2(b)), CoFe2O4 ferrite phase and BaTiO3 perovskite phase are basically formed whilst some BaCO3 still exists, which is verified by the above FTIR analysis (Fig.1(c)). When the calcination temperature increases up to 900 ℃ (Fig.2(c), the reflections are indexed just to both CoFe2O4 and BaTiO3 phases. With the calcination temperature increasing from 900 to 1 180 ℃ (Figs.2(c)-(f)), the diffraction peaks for the CoFe2O4 and BaTiO3 phases correspondingly become narrower and sharper, which means that the crystallites building the composite fibers gradually increase and the crystallization is improved.
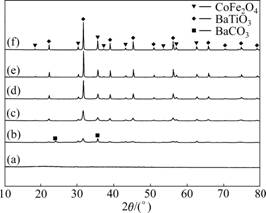
Fig.2 XRD patterns of gel precursor and 0.4CoFe2O4- 0.6BaTiO3 fibers calcined at different temperatures: (a) Gel precursor; (b) 800 ℃; (c) 900 ℃; (d) 1 000 ℃; (e) 1 100 ℃; (f) 1 180 ℃
The average crystalline grain size (D) of CoFe2O4 and BaTiO3 phases in the composite fibers can be calculated from the diffraction data in the XRD pattern using Scherrer equation. Fig.3 shows the calculated grain size D of CoFe2O4 and BaTiO3 phases in xCoFe2O4- (1-x) BaTiO3 (x=0.2, 0.3, 0.4, 0.5) fibers with the calcination temperature. It is found that the grain sizes are influenced largely by the calcination temperature. For both CoFe2O4 and BaTiO3 phases in the molar fraction range of 0.2-0.5, D values increase from 25 to 65 nm with the calcination temperature from 900 to 1 180 ℃. So, the crystalline grain sizes of CoFe2O4 and BaTiO3 in nanocomposites can be tailored by controlling the thermal decomposition process.
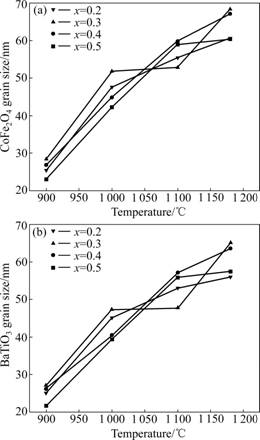
Fig.3 Grain size of xCoFe2O4-(1-x)BaTiO3 fibers with calcination temperature: (a) For CoFe2O4 grain size; (b) For BaTiO3 grain size
The SEM images of the nanocomposite 0.4CoFe2O4-0.6BaTiO3 fibers obtained at 1 180 ℃ are shown in Fig.4. The fibers (Fig.4(a)) are characterized with a diameter range of 1-7 mm, a high aspect ratio up to 1×105 and a dense surface. The morphology of a single nanocomposite fiber is shown in Fig.4(b), which is constructed by nanoparticles. These nanoparticles of CoFe2O4 and BaTiO3 are arranged along the fiber with a necklace-like structure, which allows a maximum magnetoelectric coupling without a substrate constraint and magnify the mechanical displacement arising from the piezoelectric or magnetostrictive effect due to a high aspect ratio [13]. The morphologies of other nanocomposites xCoFe2O4-(1-x)BaTiO3 fibers with various molar fractions (x=0.2, 0.3, 0.5) are very similar.
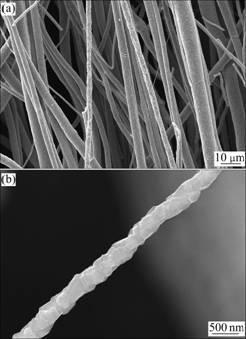
Fig.4 SEM images of 0.4CoFe2O4-0.6BaTiO3 fibers: (a) Multi- fibers; (b) Single fiber
3.3 Magnetic properties
The hysteresis loops were measured to determine the saturation magnetization (Ms) and coercivity (Hc). Fig.5 shows the hysteresis loops of the randomly oriented nanocomposite 0.4CoFe2O4-0.6BaTiO3 fibers obtained at different calcination temperatures for 2 h. These hysteresis loops exhibit soft magnetic characteristics of CoFe2O4 ferrites, implying a magnetic ordering in these nanocomposites. The magnetic parameters of 0.4CoFe2O4-0.6BaTiO3 fibers with calcination temperature are represented in Table 1. It can be observed that Ms increases from 21.5 to 35.5 A?m2/kg as the calcination temperature increases from 900 to 1 180 ℃. This phenomenon may be attributed to the crystallization improvement and grain growth, which can reduce the crystal defects and enhance a net magnetic moment [22].
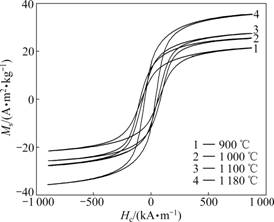
Fig.5 Hysteresis loops of randomly oriented 0.4CoFe2O4- 0.6BaTiO3 fibers obtained at different calcination temperatures
As shown in Table 1, the coercivity initially increases with the increase of the calcination temperature, reaches a maximum value of 88.4 kA/m at 1 000 ℃, and then decreases with the further increase of calcination temperature. For the randomly oriented nanocomposite CoFe2O4-BaTiO3 fibers, the coercivity should be primarily due to the magnetocrystalline anisotropy but the shape anisotropy. According to the STONER- WOHLFARTH single domain theory [23], the magnetocrystalline anisotropy energy of a nanocrystal single-domain is proportion to the nanocrystal particle volume. At low calcination temperatures the average crystallite size of CoFe2O4 in CoFe2O4-BaTiO3 fibers is supposed within the critical single-domain size, and the magnetocrystalline anisotropy increases with the increase of the grain size. With a further increase of calcination temperature over 1 000 ℃, the coercivity exhibits a reduction tendency as the CoFe2O4 grain size is larger than the critical single-domain size, and the grains become multi-domains. The domain-wall movements taking place in these multi-domain grains result in the coercivity reduction [24]. It can be deduced that the critical single-domain size of CoFe2O4 grains in the 0.4CoFe2O4-0.6BaTiO3 fibers is around 45 nm, which is close to 40 nm for the CoFe2O4 nanoparticles reported by CHINNASAMY et al [25].
Table 1 Effect of calcination temperature on CoFe2O4 grain size (D) and magnetic properties of 0.4CoFe2O4-0.6BaTiO3 fibers
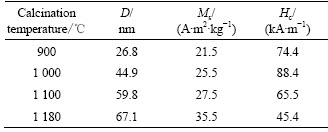
Fig.6 shows the hysteresis loops of the randomly oriented nanocomposite xCoFe2O4-(1-x)BaTiO3 (x=0.2, 0.3, 0.4, 0.5) fibers obtained at 1 180 ℃ for 2 h. Based on the magnetic properties estimated from the hysteresis loop measurements, the relationships between saturation magnetization (Ms) and remanent magnetization (Mr) of these nanocomposite CoFe2O4-BaTiO3 fibers with the molar fraction of CoFe2O4 ferrite phase are plotted in Fig.7. It can be seen that Ms and Mr show almost a linear relationship with the molar fraction of CoFe2O4 ferrite, which implies a high magnetic ordering arising from the CoFe2O4 ferrite in these nanocomposite fibers, and a tight coupling between the ferromagnetic CoFe2O4 ferrite and ferroelectric BaTiO3 perovskite can be expected. The magnetoelectric effect for these nanocomposite CoFe2O4-BaTiO3 fibers is under investigation.
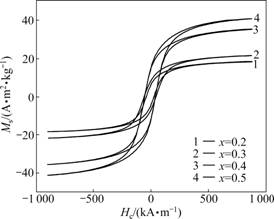
Fig.6 Hysteresis loops of randomly oriented xCoFe2O4- (1-x)0.6BaTiO3 fibers obtained at 1 180 ℃ for 2 h
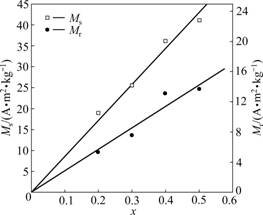
Fig.7 Ms and Mr of xCoFe2O4-(1-x)BaTiO3 fiber with molar fraction (x) of CoFe2O4
4 Conclusions
(1) Nanocomposite xCoFe2O4-(1-x)BaTiO3 (x=0.2, 0.3, 0.4, 0.5) fibers are prepared by the organic gel-thermal decomposition process using citric acid and metal salts. These fibers are composed of nanosized ferrite CoFe2O4 and ferroelectric BaTiO3 and have diameters as thin as 1 ?m, aspect ratios as high as 1× 105 and a dense surface.
(2) The average grain sizes of CoFe2O4 and BaTiO3 in the nanocomposite fibers increase from 25 to 65 nm with the calcination temperature increasing from 900 to 1 180 ℃. These nanosized grains fabricate the single nanocomposite fiber with a necklace-like structure.
(3) The magnetic properties for the nanocomposite fibers are largely influenced by the grain size and molar fraction of CoFe2O4 ferrite phase.
References
[1] IIJIMA S. Helical microtubules of graphitic carbon [J]. Nature, 1991, 354: 56-58.
[2] MORALES A M, LIEBER C M. A laser ablation method for the synthesis of crystalline semiconductor nanowires [J]. Science, 1998, 279(5348): 208-211.
[3] MARTIN C R. Nanomaterials: A membrane-based synthetic approach [J]. Science, 1994, 266(5193): 1961-1966.
[4] HAN Wei-qiang, FAN Shou-shan, LI Qun-qing, HU Yong-dan. Synthesis of gallium nitride nanorods through a carbon nanotube- confined reaction [J]. Science, 1997, 277(5330): 1287-1289.
[5] PAN Zheng-wei, DAI Zu-rong, WANG Zhong-lin. Nanobelts of semiconducting oxides [J]. Science, 2001, 291(5510): 1947-1949.
[6] JU Y W, PARK J H, JUNG H R, CHO S J, LEE W J. Electrospun MnFe2O4 nanofibers: preparation and morphology [J]. Composites Science and Technology, 2008, 68(7/8): 1704-1709.
[7] CAO Kai, SHEN Xiang-qian, JING Mao-xiang, ZHOU Jian-xin. Preparation of ferromagnetic metal fine fibers by organic gel-thermal reduction process [J]. Journal of Central South University of Technology, 2007, 14(5): 607-611.
[8] WANG Wen-zhong, LIU Ying-kai, XU Cong-kang, ZHENG Chang-lin, WANG Guang-hou. Synthesis of NiO nanorods by a novel simple precursor thermal decomposition approach [J]. Chemical Physics Letters, 2002, 362(1/2): 119-122.
[9] ZHANG Chun-ye,SHEN Xiang-qian,ZHOU Jian-xin, JING Mao- xiang, CAO Kai. Preparation of spinel ferrite NiFe2O4 fibres by organic gel-thermal decomposition process [J]. Journal of Sol-Gel Science and Technology, 2007, 42(1): 95-100.
[10] XIANG Jun, SHEN Xiang-qian, MENG Xian-feng. Preparation of Co-substituted MnZn ferrite fibers and their magnetic properties [J]. Materials Chemistry and Physics, 2009, 114: 362-366.
[11] SRINIYAS S, LI J Y. The effective magnetoelectric coefficients of polycrystalline multiferroic composites [J]. Acta Materialia, 2005, 53: 4135-4142.
[12] HUA Z, YANG P, HUANG H. Sol-gel template synthesis and characterization of magnetoelectric CoFe2O4/Pb(Zr0.52Ti0.48)O3 nanotubes [J]. Materials Chemistry and Physics, 2008, 107: 541-546.
[13] XIE S H, LI J Y, LIU Y Y, LAN L N, JIN G, ZHOU Y C. Electrospinning and multiferroic properties of NiFe2O4- Pb(Zr0.52Ti0.48)O3 composite nanofibers [J]. Journal of Applied Physics, 2008, 104: 024115.
[14] XIAO Shun-hua, JIANG Wei-fen, LI Long-yu, LI Xin-Jian. Low-temperature auto-combustion synthesis and magnetic properties of cobalt ferrite nanopowder [J]. Materials Chemistry and Physics, 2007, 106: 82-87.
[15] ZHANG Shu-ping, DONG Da-wei, SUI Yu, LIU Zhi-guo, WANG Hong-xia, QIAN Zheng-nan, SU Wen-hui. Preparation of core shell particles consisting of cobalt ferrite and silica by sol-gel process [J]. Journal of Alloys and Compounds, 2006, 415: 257-260.
[16] RAMAJO L, PARRA R, REBOREDO M. Heating rate and temperature effects on the BaTiO3 formation by thermal decomposition of (Ba,Ti) organic precursors during the Pechini process [J]. Materials Chemistry and Physics, 2008, 107: 110-114.
[17] RADWAN N R E, ELSHOBAKY H G. Solid-solid interactions between ferric and cobalt oxides as influenced by Al2O3-doping [J]. Thermochimica Acta, 2000, 360: 147-156.
[18] WANG Li-qiu, LIU Liang, XUE Dong-feng. Wet routes of high purity BaTiO3 nanopowders [J]. Journal of Alloys and Compounds, 2007, 440: 78-83.
[19] GARCIA C L A, MONTEMAYOR S M. Synthesis of CoFe2O4 nanoparticles embedded in a silica matrix by the citrate precursor technique [J]. Journal of Magnetism and Magnetic Materials, 2005, 294: e43-e46.
[20] RAMAJO L, CASTRO M S, REBOREDO M M. Effect of silane as coupling agent on the dielectric properties of BaTiO3-epoxy composites [J]. Composites (Part A): Applied Science and Manufacturing, 2007, 32: 1852-1859.
[21] KASAPOGLU N, BAYKAL A, KOSEOGLUAND Y. Microwave-assisted combustion synthesis of CoFe2O4 with urea, and its magnetic characterization [J]. Scripta Materialia, 2007, 57: 441-444.
[22] CHIU W S, RADIMAN S, ABDSHUKOR R, ABDULLAH M H, KHIEW P S. Tunable coercivity of CoFe2O4 nanoparticles via thermal annealing treatment [J]. Journal of Alloys and Compounds, 2008, 459: 291-297.
[23] STONER E C, WOHLFARTH E P A. Mechanism of magnetic hysteresis in heterogeneous alloys [J]. IEEE Transactions on Magnetics, 1991, 27(4): 3475-3518.
[24] MAAZ K, MUMTAZ A, HASANSIN S K, CEYLAN A. Synthesis and magnetic properties of cobalt ferrite (CoFe2O4) nanoparticles prepared by wet chemical route [J]. Journal of Magnetism and Magnetic Materials, 2007, 308: 289-295.
[25] CHINNASAMY C N, JEYADEVAN B, SHINODA K, TOHJI K, DJAYAPRAWIRA D J, TAKAHASHI M, JOSEYPHUS R J, NARAYANASAMY A. Unusually high coercivity and critical single-domain size of nearly monodispersed CoFe2O4 nanoparticles [J]. Applied Physics Letters, 2003, 83: 2862-2864.
(Edited by CHEN Wei-ping)
Foundation item: Project(50674048) supported by the National Natural Science Foundation of China; Project(20080431069) supported by China Postdoctoral Science Foundation; Project(CX10B-257Z) supported by Postgraduate Cultivation and Innovation Foundation of Jiangsu Province, China
Received date: 2009-12-12; Accepted date: 2010-04-08
Corresponding author: SHEN Xiang-qian, PhD, Professor; Tel: +86-511-88791964; E-mail: shenxq@ujs.edu.cn