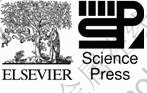
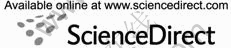
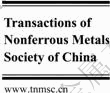
Trans. Nonferrous Met. Soc. China 22(2012) 2007-2013
Direct electrochemical preparation of CeCo5 alloy from mixed oxides
DAI Lei1,2, WANG Shuo1, LI Yue-hua1, WANG Ling1, SHAO Guang-jie2
1. College of Chemical Engineering, Hebei United University, Tangshan 063009, China;
2. College of Environmental and Chemical Engineering, Yanshan University, Qinhuangdao 066004, China
Received 24 October 2011; accepted 4 May 2012
Abstract: CeCo5 alloy was prepared from the mixture of cobalt oxide (Co3O4) and cerium oxide (CeO2) powders by electro-deoxidation in molten calcium chloride. The effects of the cell voltage and sintering temperature on the electrolysis process were reported. The electro-deoxidation mechanism was investigated by potentiodynamic polarization using a molybdenum cavity electrode in conjunction with characterization of the products from constant voltage electrolysis under different conditions by XRD and SEM. The electrochemical property of CeCo5 alloy was investigated by cyclic voltammetry measurements. The results show that the electro-deoxidation rate increases with increasing the cell voltage and decreasing the sintering temperature. The pure CeCo5 can be prepared by direct electro-deoxidation of mixed CeO2/Co3O4 pellets sintered at 850 ℃ when the cell voltage of 3.1 V is applied. The electro-deoxidation proceeds by the simultaneous reduction of Co3O4 to Co and reduction of CeO2 to CeOCl, followed by CeOCl reduction on the pre-formed Co to form CeCo5 alloy which shows a good cycling stability.
Key words: electro-deoxidation; CeCo5 alloy; CaCl2 melt; oxides
1 Introduction
AB5 alloys of rare earth and d-metal with CaCu5 type structure are often considered to be the most promising electrode materials for Ni-MH batteries applications due to their high hydrogen absorption capacity, the favorable electrochemical activation as well as improved charge and discharge kinetic properties [1-3]. The commonly used preparation process for above alloys is the induction melting method, which involves separate extraction and refining the individual metals of high purity, followed by a prolonged annealing process at elevated temperatures. It is obvious that the production process is long and the cost is high [4]. An alternative production method is necessary to create low-cost AB5 alloys.
The FFC-Cambridge process, which is a direct electro-deoxidation of solid oxides to metals and alloys in molten salts, seems to be the alternative process due to its simplicity and less investment [5]. Compared with other extractive metallurgical processes, the main perceived advantages of the FFC-Cambridge process include a relatively low energy consumption, relatively simple operation, and capability to directly reduce a combination of different metal oxides together to form alloys [6-8]. A significant number of laboratory studies have been concentrated on electrochemical synthesis of numerous metals and alloys directly from their metal oxides [9-12].
In the present work, applying the FFC-Cambridge process, hydrogen storage alloy CeCo5 was prepared directly from mixed CeO2/Co3O4 powders. The products from electrolysis under different conditions were analyzed by XRD and SEM. The mechanism of the electro-deoxidation was proposed based on experimental evidences.
2 Experimental
2.1 Pellet preparation
Commercially available powders of cerium oxide (CeO2) and cobalt oxide (Co3O4) were used as raw materials. The above oxide powders were weighed according to the stoichiometric ratio of CeCo5 and then polyvinyl butyral (PVB), (1% in mass fraction) and acetone were added to the mixture and thoroughly milled in a ball-milling container for 3 h. After drying, the mixture was pressed under 30 MPa into pellets of 10 mm in diameter and 3 mm in thickness. The pellets were calcined in air at 400 ℃ for 4 h to remove the PVB and then were sintered at 850, 1050 and 1250 ℃ for 5 h, respectively. The sintered pellets were characterized by X-ray diffraction with Cu Kα radiation (XRD, Vantage 4.0) and scanning electron microscope (SEM, S°4800). Anhydrous CaCl2 and a high pure graphite (99.9%) were used for the electrolysis. Argon gas (99.99%) was used as the protective gas during high temperature experiments.
2.2 Constant voltage electrolysis
The experimental apparatus used here was similar to that described in Ref. [13]. An alumina crucible was filled with about 800 g of a dehydrated CaCl2 and placed at the bottom of a stainless steel tube reactor in a vertical furnace. A thermocouple was placed between the alumina crucible and the inner wall of the stainless steel reactor. Subsequently, argon gas was introduced into the reactor continuously. The temperature was increased and kept the pre-set temperature. Electrolysis of the sandwich oxide cathode with one or two pellets proceeded at a constant voltage with high pure graphite used as the counter electrode. The experiment data were collected by a PC computer aid system. After electrolysis, the sample was lifted from the molten salt and cooled naturally in a stream of argon before removing from the steel reactor, and then was immediately washed in distilled water. The composition and morphology of the products were analyzed by XRD and SEM, respectively.
2.3 Potentiodynamic polarization
Foils of molybdenum (width of 10 mm, thickness of 0.5 mm, length of 20 mm, and purity of 99.9%) were used as the substrate for making the metallic cavity electrodes (MCE). One circular holes (1.0 mm in diameter) were drilled through the foils by mechanical drill. The oxide powders, such as CeO2 powder, Co3O4 powder and mixed CeO2 and Co3O4 powders (molar ratio of Ce toCo of 1:5) were manually filled into the MCE cavity by repeatedly finger-pressing and used as the working electrode on which cathodic polarization was then carried out potentiodynamically with 1 mV/s of sweep rate in molten CaCl2 at 850 ℃. A high pure graphite (99.9%) was used as the counter electrode. The Kanthal wire was used as the pseudo-reference electrode whose stability was satisfactory [14]. All electrochemical experiments were operated under the protection of an argon flow. Polarization curves were then recorded on ZAHNER IM6e electrochemical station.
2.4 Measurement of electrochemical property
The electrochemical property of CeCo5 alloy was investigated by cyclic voltammetry experiments in a classical three-electrode cell and the CeCo5 alloy powder microelectrode was used as working electrode. A Pt plate and a Hg/HgO electrode were used as counter electrode and reference electrode, respectively. The cyclic voltammogram curves were measured at the rate of 50 mV/s from -1100 mV to -300 mV (versus Hg/HgO electrode) in a 6 mol/L KOH solution.
3 Results and discussion
3.1 Influence of cell voltage
The appropriate cell voltages are needed for the electro-deoxidation process to avoid salt decomposition but achieve relatively high electro-deoxidation speed [15,16]. The theoretical decomposition voltages of Co3O4,CeO2 and CaCl2 at 850 ℃ are listed in Table 1. To avoid the decomposition of CaCl2, the cell voltage during the electro-deoxidation of the mixed oxide pellets should be controlled at less than 3.2 V. Figure 1 presents typical current—time plots recorded during electrolysis of the oxide pellets in molten CaCl2 at 850 ℃ at different cell voltages for 20 h. The I—t plots at 2.1, 2.8 and 3.1V exhibit similar features. The current declines with time at beginning, reaching a trough value in the first few minutes, which may correspond to the electrolytic cell to reach equilibrium, and then the current increases to a peak. The increase of the current to a peak is attributed to the expansion of three-phase interlines (3PIs, metal/ oxide/electrolyte) [17]. When the oxide in contact with the Kanthal wire is reduced to metal, new 3PIs form amongst the newly formed metal/oxide/electrolyte. With processes continuing, the 3PIs expand, which increases the contact area of the metallic current collector. With the 3PIs moving into the interior of the oxide pellet along the depth direction, the current declines continuously, which is mostly because of mass transfer difficulties, and the background current is due to electronic conduction through molten CaCl2, which may result from the drop of the graphite particles and the impurity of the molten salt [18,19]. It can be seen that the current increases with increasing the cell voltage, which indicates a higher reduction rate at the cell voltage of 3.1 V.
Table 1 Theoretical decomposition voltage of Co3O4, CeO2 and CaCl2 at 850 ℃
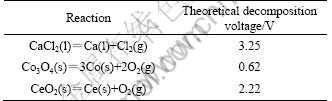
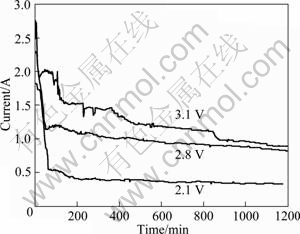
Fig.1 Current—time plots of electrolysis of pellets (sintering at 850 ℃ in air for 5 h) at different cell voltages for 20 h in CaCl2 molten salt at 850 ℃
The electrolysis products were analyzed by XRD and the spectra are shown in Fig. 2. When 2.1 V was applied, the XRD pattern of the product exhibits the CeOCl, Co and CeO2 phases, but no CeCo5 phase is formed. At 2.8 V, in the XRD pattern of the product only CeOCl and Co peaks appear. It can be concluded that the mixed oxides only can be reduced to Co and CeOCl at 2.8 V, and the formation of CeCo5 needs a higher cell voltage, which is due to the individual difficulty of the electro-deoxidization of CeO2 (see Table 1). The XRD pattern of the product after electrolysis at 3.1 V shows that pure CeCo5 phase is formed.
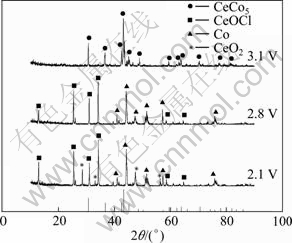
Fig. 2 XRD patterns of products from electrolysis of pellets (sintered at 850 ℃ in air for 5 h) at different cell voltages for 20 h in CaCl2 melt at 850 ℃
3.2 Influence of sintering temperature
It has been claimed that the sintering temperature of the pellets plays an important role in the electro-deoxidization process [20]. In order to study the influence of sintering temperature, the oxide pellets were sintered at 850, 1050 and 1250 ℃ for 5 h in air. The porosity and density of the pellets after being sintered at three different temperatures are shown in Table 2. The data demonstrate that varying the sintering temperatures from 850 to 1050 ℃ influences very little on the apparent densities and porosities of the pellets. When the pellets were sintered at 1250 ℃, the decrease of the porosity and increase of the density are remarkable, which may lead to decrease of electrolysis rate. Figure 3 shows the microstructures of the cross section of the pellets sintered at 850, 1050 and 1250 ℃, prior to electrolysis. It can be seen that the microstructures of the pellets sintered at 850 ℃ and 1050 ℃ are similar to the pellet before sintering. But sintering of the pellets involves both the growth of strength and the shrinkage of the pellets, which can avoid powdering of the pellets in the molten. When the pellets were sintered at 1250 ℃, a mass of particles were clearly seen and their particle size was in the range of 1-10 μm.
Table 2 Porosity and density of pellets after sintering at three different temperatures
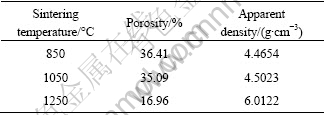
Figure 4 shows the XRD patterns of mixed oxides sintered at 850, 1050 and 1250 ℃ in air for 5 h. The pellets sintered at 850 ℃ still consist of the original oxides of CeO2 and Co3O4 and no new oxides appear, which means that the pellets are stable at below 850 ℃. After being sintered at 1050 ℃ and 1250 ℃, CoO appears, which is caused by the decomposition of Co3O4 [21].
The typical constant voltage chronoamperograms of electrolysis of the pellets sintered at different temperatures are shown in Fig. 5. With increasing the sintering temperature, the current becomes low. It is understood that the electro-deoxidization involves oxygen ion diffusion through the oxide/electrolyte interface. When the pellets were sintered at higher temperatures, the increase of the extent of the densification means the decrease of interface areas, which results in a slower reduction rate.
Figure 6 displays the XRD patterns of the samples sintered at 850, 1050, 1250 ℃ and then electrolyzed at 3.1 V for 20 h. As seen in Fig. 6, the sample sintered at 850 ℃ is completely electrolyzed and pure CeCo5 phase is formed. As to the pellets sintered at 1050 ℃, XRD spectrum shows that the product mainly consists of CeCo5, but a little CeOCl and Co phases exist. The sample sintered at 1250 ℃ after electrolysis shows that some CeCo5 is formed, but CeOCl and Co phases are dominant. Clearly, the results further demonstrate that increasing the sintering temperature does lead to a lower reduction rate.
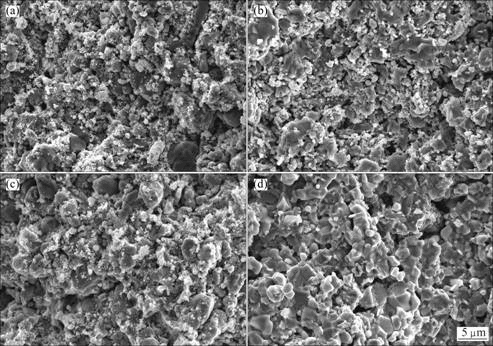
Fig. 3 SEM images of CeO2/Co3O4 pellets before (a) and after sintering for 5 h at 850 ℃ (b), 1050 ℃ (c) and 1250 ℃ (d)
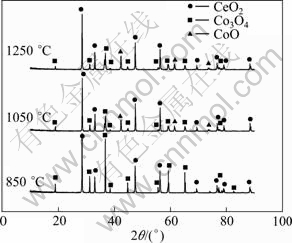
Fig. 4 XRD patterns of mixed CeO2/Co3O4 pellets sintered at different temperatures for 5 h
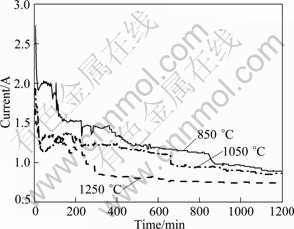
Fig. 5 Current—time plots of electrolysis of pellets sintered at different temperatures for 5 h and then electrolyzed at 3.1 V for 20 h
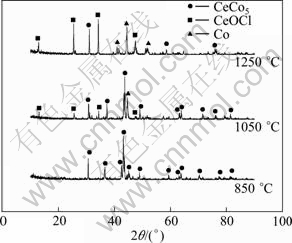
Fig. 6 XRD patterns of products from electrolysis of pellets sintered at different temperatures and then electrolyzed at 3.1 V for 20 h
3.3 Influence of electrolysis time
In order to investigate the effect of electrolysis time on the electro-deoxidation process, the electrolysis was carried out at 3.1 V for different time and then the obtained products were analyzed by XRD. Figure 7 shows the XRD patterns of the products that were electrolyzed at 3.1 V for 1, 7, 12 and 20 h. After being electrolyzed for 1 h, the XRD pattern of the product exhibits CoO, CeO2, CeOCl and Co phases, but formation of CeCo5 phase does not take place, which may suggest that reactions (1), (2) and (3) occur, that is, the Co3O4 reduces to CoO and Co, and the CeO2 reduces to CeOCl.
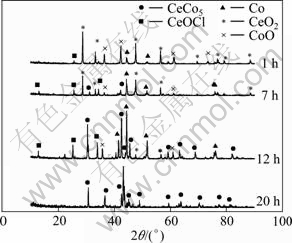
Fig. 7 XRD patterns of products from electrolysis of pellets (sintered at 850 ℃ in air for 5 h) at 3.1 V for different time in CaCl2 molten salt at 850 ℃
Co3O4+2e=3CoO+O2- (1)
CoO+2e=Co+O2- (2)
CeO2+CaCl2+e=CeOCl+CaO+Cl- (3)
After 7-12 h electrolysis, the product still contains CoO, CeO2, CeOCl and Co phases, but CeCo5 alloy appears. With prolonging the electrolysis time, the amount of Co3O4 gradually increases and other phases decrease. These results verify that Co3O4 reduction takes place to Co and then CeOCl is reduced on the newly-formed Co surface to form the alloy that can be expressed as reaction(4). After electrolysis for 20 h, pure CeCo5 alloy can be obtained, which shows that in the course of electrolysis the mixture of Co3O4 and CeO2 is completely deoxidized.
5Co+CeOCl+3e=CeCo5+O2-+Cl- (4)
Figure 8 shows SEM images of the electrolysis products after electrolyzing for different time. The SEM image of the product electrolyzed for 1 h, as seen in Fig. 8(a), is similar to the pre-electrolyzed one in Fig. 3(b), which shows compact structure, but the particle size seems to be large. After 7-12 h electrolysis, the pellets become porous and the particles seem irregular (shown in Figs. 8(b), (c)). After electrolysis for 20 h, as seen in Fig. 8(d), the precursor was transformed into final product which shows spongy and porous with the nodular particles. The reduced pellets can easily be ground into powder with pestle and mortar.
3.4 Potentiodynamic polarization
To further understand the electro-deoxidation process mechanism, the cathodic polarization behavior of samples on the MCE were measured in a molten CaCl2 at 850 ℃. Figure 9 shows the cathodic polarization curves of pure CeO2 powder, pure Co3O4 powder and mixed CeO2 and Co3O4 powders recorded in molten CaCl2 at 850 ℃. In the absence of oxide, the polarization curve of the bare MCE shows a reduction peak c2 formed at -1.2 V which can be attributed to the reduction of CaCl2 [13,15]. The polarization feature of the pure CeO2 powder at 0 to -0.6 V is similar to that of the bare MCE. Then the current becomes larger than that of the bare MCE until the peak c2 appears, which may suggest in reaction (3) that the CeO2 reduces to CeOCl. The polarization curve of the pure Co3O4 powder exhibits a reduction peak c1 at -0.23 V which does not appear in polarization curves of the bare MCE and the pure CeO2 powder. This peak c1 can be attributed to the reduction of Co3O4 into Co metal. The polarization features of the mixed CeO2 and Co3O4 powders are similar to those observed on the polarization curve of the pure Co3O4 powder, but value of the reduction peak c1 is larger than that of the pure Co3O4 powder, which is likely caused by the formation of the Ce-Co compound resulting from the reduction of CeO2 or CeOCl on the newly formed Co metal particles.
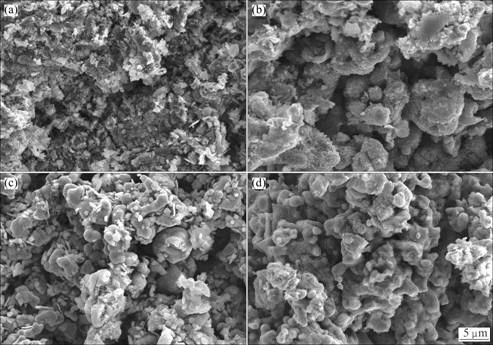
Fig. 8 SEM images of products from electrolysis of pellets (sintered at 850 ℃ in air for 5 h) at 3.1 V for different time in CaCl2 molten salt at 850 ℃: (a) 1 h; (b) 7 h; (c) 12 h; (d) 20 h
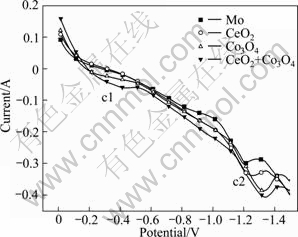
Fig. 9 Cathodic polarization curves of MCE in the absence and presence of CeO2 powder, Co3O4 powder and mixed CeO2 and Co3O4 powders in CaCl2 molten salt at 850 ℃ (Scan rate: 1 mV/s)
3.5 Electrochemical property of CeCo5 powders
Cyclic voltammetric measurements were done in the potential range of -1100 to -300 mV (versus Hg/HgO electrode) to investigate the electrochemical property of CeCo5 powders. Figure 10 illustrates the cyclic voltammograms of the CeCo5 alloy microelectrode. The number on the curves represents the cycle number. The anodic peak observed is due to the oxidation of the desorbed hydrogen atoms on the surface, which is used for evaluating the discharge processes of the CeCo5 alloy. The discharge capacities were calculated based on the anodic peak areas after different cycles, as shown in Fig. 11. The activation of CeCo5 is almost complete after 50 cycles and the maximum discharge capacity obtained is 183 mA·h/g. It can be seen that after 140 cycles the capacity retention is approximately 92.8%.
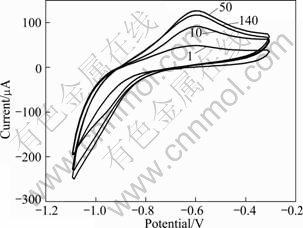
Fig. 10 Voltammogram curves of CeCo5 alloy microelectrode in 6 mol/L KOH solution (potential scanning rate: 50 mV/s)
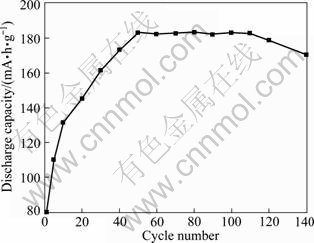
Fig. 11 Discharge capacity of CeCo5 alloy versus cycle number
4 Conclusions
The hydrogen storage alloy CeCo5 can be prepared via direct electro-deoxidation of sintered pellets of mixed CeO2/Co3O4 powders sintered at 850 ℃ in molten CaCl2 when 3.1 V was applied. As to the pellets electrolyzed at lower cell voltages, the product includes CeOCl, Co and CeO2, but no CeCo5 phase. Raising the sintering temperature leads to the decrease in the porosity and increase in the extent of the densification, which decreases the electro-deoxidation rate. The mechanism of the electro-deoxidation process was observed using XRD and potentiodynamic polarization, which was found to be split into two steps: the reduction on the mixed oxide starts from Co3O4 to Co and CeO2 to CeOCl, followed by that of CeOCl on the surface of newly-formed Co to form the CeCo5 alloy. The CeCo5 alloy has a maximum discharge capacity of 183 mA·h/g and the capacity retention is approximately 92.8% after 140 cycles.
References
[1] TLIHA M, BOUSSAMI S, MATHLOUTHI H, LAMLOUMI J. Percheron-Guégan A. Electrochemical characteristics of AB5-type hydrogen storage alloys [J]. Journal of Alloys and Compounds, 2010, 506: 559-564.
[2] SLEPSKI P, DAROWICKI K, KOPCZYK M, SIERCZYNSKA A, ANDREARCZYK K. Electrochemical impedance studies of AB5-type hydrogen storage alloy [J]. Journal of Power Sources, 2010, 195: 2457-2462.
[3] SRIVASTAVA S, UPADHYAYA R K. Investigations of AB5-type hydrogen storage materials with enhanced hydrogen storage capacity [J]. International Journal of Hydrogen Energy, 2011, 36: 7114-7121.
[4] RONG Q, SCHALLER H J. On the constitution and thermodynamics of Ni–Tb alloys [J]. Journal of Alloys and Compounds, 2004, 365: 188-196.
[5] CHEN G Z, FRAY D J, FARTHING T W. Direct electrochemical reduction of titanium dioxide to titanium in molten calcium chloride [J]. Nature, 2000, 407: 361-364.
[6] GLOWACKI B A, FRAY D J, YAN X Y, CHEN G Z. Superconducting Nb3Sn intermetallics made by electrochemical reduction of Nb2O5–SnO2 oxides [J]. Physica C, 2003, 387: 242-246.
[7] SONG Qiu-shi, XU Qian, KANG Xue, DU Ji-hong, XI Zheng-ping. Mechanistic insight of electrochemical reduction of Ta2O5 to tantalum in a eutectic CaCl2–NaCl molten salt [J]. Journal of Alloys and Compounds, 2010, 490: 241-246.
[8] CLAUX B, SERP J, FOULETIER J. Electrochemical reduction of cerium oxide into metal [J]. Electrochimica Acta, 2011, 56: 2771-2780.
[9] XIAO Wei, JIN Xian-bo, DENG Yuan, WANG Di-hua, CHEN G Z. Rationalisation and optimisation of solid state electro-reduction of SiO2 to Si in molten CaCl2 in accordance with dynamic three-phase interlines based voltammetry [J]. Journal of Electroanalytical Chemistry, 2010, 639: 130-140.
[10] LI Guo-ming, JIN Xian-Bo, WANG Di-hua, CHEN G Z. Affordable electrolytic ferrotitanium alloys with marine engineering potentials [J]. Journal of Alloys and Compounds, 2009, 482: 320-327.
[11] WANG Di-hua, QIU Guo-hong, JIN Xian-bo, HU Xiao-hong, CHEN G Z. Electrochemical metallization of solid terbium oxide [J]. Angew Chem Int Ed, 2006, 45: 2384-2388.
[12] PENG Jun-jun, ZHU Yong, WANG Di-hua, JIN Xian-bo, CHEN G Z. Direct and low energy electrolytic co-reduction of mixed oxides to zirconium-based multi-phase hydrogen storage alloys in molten salts [J]. Journal of Materials Chemistry, 2009, 19: 2803-2809.
[13] ZHAO Bing-jian, WANG Ling, DAI Lei, CUI Guang-hua, ZHOU Hui-zhu, KUMAR R V. Direct electrolytic preparation of cerium/ nickel hydrogen storage alloy powder in molten salt [J]. Journal of Alloy and Compounds, 2009, 468: 379-385
[14] GORDO E, CHEN G Z, FRAY D J. Toward optimisation of electrolytic reduction of solid chromiumoxide to chromium powder in molten chloride salts [J]. Electrochimica Acta, 2004, 49: 2195-2208.
[15] QIU Guo-hong, WANG Di-hua, JIN Xian-bo, CHEN G Z. A direct electrochemical route from oxide precursors to the terbium–nickel intermetallic compound TbNi5 [J]. Electrochimica Acta, 2006, 51: 5785-5793.
[16] ZHANG Qing-jun, QU Mei-ling, WANG Ling, DAI Lei, TIAN Ying, CUI Chun-xiang. Preparation of CoSn alloy by electro- deoxidization in molten salt [J]. The Chinese Journal of Nonferrous Metals, 2010, 20(8): 1578-1582. (in Chinese)
[17] ZOU Xing-li, LU Xiong-gang, LI Chong-he, ZHOU Zhong-fu. A direct electrochemical route from oxides to Ti-Si intermetallics [J]. Electrochimica Acta, 2010, 55: 5173-5179.
[18] DENG Yuan, WANG Di-hua, XIAO Wei, JIN Xian-bo, HU Xiao-hong, CHEN G Z. Electrochemistry at conductor/insulator/ electrolyte three-phase interlines: A thin layer model [J]. J Phys Chem B, 2005, 109: 14043-14051.
[19] QIU Guo-hong, WANG Di-hua, MA Meng, JIN Xian-bo, CHEN G Z. Electrolytic synthesis of TbFe2 from Tb4O7 and Fe2O3 powders in molten CaCl2 [J]. Journal of Electroanalytical Chemistry, 2006, 589: 139-147.
[20] JIANG Kai, HU Xiao-hong, MA Meng, WANG Di-hua, QIU Guo-hong, JIN Xian-bo, CHEN G Z. Perovskitization—Assisted lectrochemical reduction of solid TiO2 in molten CaCl2 [J]. Angew Chem Int Ed, 2006, 45: 428-432.
[21] HYSLOP D J S, ABDELKADER A M, COX A, FRAY D J. Electrochemical synthesis of a biomedically important Co–Cr alloy [J]. Acta Materialia, 2010, 58: 3124-3130.
直接电解金属氧化物制备CeCo5合金
戴 磊1, 2, 王 硕1, 李跃华1, 王 岭1, 邵光杰2
1. 河北联合大学 化学工程学院,唐山 063009;
2. 燕山大学 环境与化学工程学院,秦皇岛 066004
摘 要:在CaCl2熔盐中,以Co3O4和CeO2混合氧化物为原料,采用熔盐电脱氧法制备CeCo5合金。研究电解电压、烧结温度等对电脱氧过程的影响。采用SEM和XRD对不同条件下制备的产物微观形貌和相组成进行表征,并结合动电位极化法,研究电脱氧机理。采用循环伏安法研究材料的电化学性能。结果表明:随着电解电压的升高和烧结温度的降低,电脱氧速度逐渐加快。在850 ℃下烧结的混合氧化物试样,在3.1 V电压下电解,可制备出纯相的CeCo5合金。在电脱氧过程中,Co3O4还原成单质Co,CeO2 还原成CeOCl, CeOCl在单质Co表面还原而形成CeCo5合金。所制备的合金表现出良好的电化学循环稳定性。
关键词:电脱氧;CeCo5合金;CaCl2熔盐;氧化物
(Edited by YANG Hua)
Foundation item: Project (E2010000941) supported by Hebei Provincial Natural Science Foundation of China; Project (11212119D) supported by Hebei Provincial Science and Technology Support Program of China
Corresponding author: WANG Ling; Tel: +86-315-2592170; E-mail: tswling@126.com
DOI: 10.1016/S1003-6326(11)61421-0