
Effects of microstructure on creep behavior of Mg-5%Zn-2%Al(-2%Y) alloy
ZOU Hong-hui(邹宏辉)
General Research Institute for Nonferrous Metals, Beijing 100088, China
Received 5 June 2007; accepted 29 November 2007
Abstract: Creep tests of Mg-5%Zn-2%Al(-2%Y) (mass fraction) alloy were carried out at 175 ℃ under the load of 50 MPa, on the cast and solution condition, respectively. Relationship between the creep behavior and microstructure was studied by the optical microscopy, SEM and TEM. The result shows that the primary creep strain is relative to the thermo-stability and the quantity of intermetallic compounds in grain boundary area. During the secondary creep period, the minimum creep rate of solution-treated specimens is substantially decreased as a result of dynamic MgZn2 precipitation.
Key words: Mg alloy; microstructure; creep deformation; Mg-Zn phase; dynamic precipitation
1 Introduction
Light mass magnesium alloy receives increasing attention in industrial application, such as in the automobile and digital gadget manufacture[1-3]. However, the further application of magnesium alloy is restricted, due to its low elevated temperature properties. Mg-Al alloys such as AZ91D and AM60 can not applied to the environment where temperature surpasses 120 ℃[4-5]. Researches indicated that the deteriorated anti-creep capability of Mg-Al alloys is attributed to the solution of Al atoms, which leads to the undesirable dynamic precipitation of γ phase (Mg17Al12)[6-9].
Therefore, Mg-5%Zn-2%Al(-2%Y) (mass fraction) alloy was developed. Mg-5%Zn-2%Al alloy were designed to have high zinc contents, and Al content is lowered to be about 2%, which is far below the solubility of Al element in the magnesium matrix. About 2% yttrium was added in the Mg-5%Zn-2%Al alloy in order to promote the creep resistance. The creep behaviors and microstructures of as-cast and pre-solution treated Mg-5%Zn-2%Al(-2%Y) alloys were studied in a comparative way, in order to establish the relationship between the creep resistance capability and the thermo-stability, location, crystalline morphology of second phases in magnesium alloys with a high zinc content. The morphology and chemical composition changes of intermetallic compounds taking place during creep deformation were also studied in this work.
2 Experimental
Alloys were designed to have a nominal chemical composition of 5%Zn, 2%Al, x%Y (x=0 and 2.0) (mass fraction), which are designated as ZA52 and ZA52Y2 alloys in the work. Their chemical compositions inspected by ICP method are listed in Table 1. Commercial pure Mg, Zn, Al blocks and Mg-20%Y interalloy were molten in the experiment. Polished magnesium blocks were heated to 730 ℃ and kept at that temperature till the complete melting down. Then, polished Al, Zn chips were added into the melt, and the melt was stirred thoroughly. As for ZA52Y2 alloy, Mg-20%Y master alloy was added when the temperature of the melt reaches 780 ℃. Then, the melt was cooled and kept at 720 ℃ for about 30 min. Finally, the melt was poured into iron die preheated to 250 ℃. The whole process was conducted under the protection of SF6 and CO2 mixed atmosphere (φ(SF6)?φ(CO2)=1?100). Solution treatments were carried out at 340 ℃ for 24 h, with SO2 employed as a protective gas. The microstructures of two alloys were revealed by 4% Nital solution, and etching time was about 20 s. Further SEM analysis was conducted, and EDAX analysis was performed to determine the phase constitution. The creep tests of as-cast and pre-solution treated specimens were carried out at 175 ℃ under the pressure of 50 MPa. Also, some specimens were tested to study the interaction between dynamic precipitates and dislocations. Small discs used for TEM observation were sampled from thin slices, which were sectioned from the middle part of these creep specimens, with section plane parallel to the loading direction. Slices were mechanically ground to less than 0.1 mm in thickness, and punched to discs with a diameter of 3 mm. Then, the discs were twinjet electropolished in a solution of 1% perchloric acid- ethanol solution at -55 ℃ and 0.1 A. The observation was made on the JEOL 2010 model TEM, with a accelerating voltage of 160 kV.
Table 1 Chemical compositions of studied alloys

3 Results
3.1 Microstructure of as-cast and pre-solution treated ZA52 and ZA52Y2 alloys
The microstructures of as-cast ZA52 alloy under the OM and SEM are revealed in Figs.1(a) and 1(c), respectively. It are mainly composed of α matrix and divorced eutectic phase, and divorced eutectic phase distributes in the boundary area. EDAX analysis indicates that the eutectic lumps are mainly composed of Mg and Zn elements, and a tiny fraction of Al element is also found to dissolve into them. The ratio of the amount of the substrate, n(Mg)?n(Zn)?n(Al)≈50.8?20.8?1.9. Therefore, the eutectic phase is binary Mg51Zn20 phase. Two new phases form in the as-cast microstructure of ZA52Y2 alloy, as a result of the addition of about 2% yttrium (Figs.1(b) and 1(d)). The coarse polygon particles are identified as Al2Y phase by EDAX method, and the branch-structure phase located at the boundary area is proved to be Al11Y3 intermetallic compound. Mg51Zn20 eutectic phase is also found to be included in the microstructure, but it no longer appears as a dominant phase on the grain boundary of ZA52Y2 alloy.
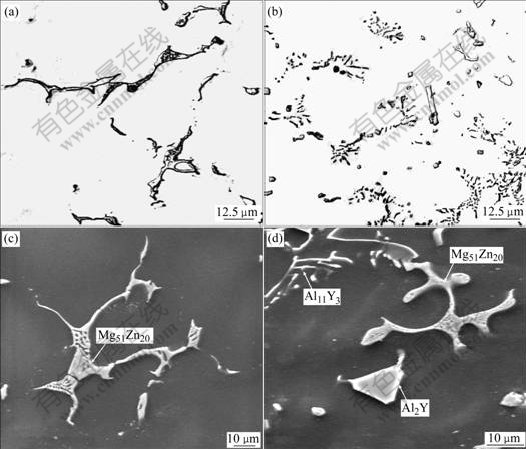
Fig.1 As-cast microstructures of alloys: (a) ZA52 alloy under OM; (b) ZA52Y2 under OM; (c) ZA52 alloy under SEM; (d) ZA52Y2 alloy under SEM
After solution at 340 ℃ for 24 h, Mg-Zn eutectic phase can hardly be observed in the microstructure of ZA52 and ZA52Y2 alloys under the optical microscope, as seen in Fig.2. Some Al11Y3 phase and Al2Y phase remain in the solution treated ZA52Y2 alloy.
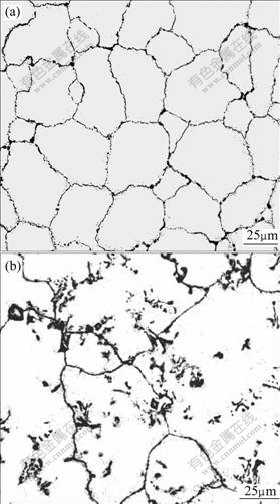
Fig.2 Microstructures of solution treated ZA52(a) and ZA52Y2(b) alloys (340 ℃, 24 h)
3.2 Creep behaviors of as-cast and pre-solution treated alloys
The creep curves of as-cast and pre-solution treated ZA52 and ZA52Y2 alloys are shown in Figs.3(a) and 3(b), respectively. The minimum creep rate, primary creep strain and the total creep strain of studied specimens are listed in Table 2. For ZA52 alloy, its as-cast specimen has a relatively small creep rate during the primary period, and secondary creep period starts at about 19 h. However, the pre-solution treated specimen exhibits a larger creep rate during the primary period, but it has a smaller minimum creep rate during the secondary period. The 100 h creep strain is almost the same for both cast and pre-solution-treated ZA52 alloy. Almost the same tendency is repeated for ZA52Y2 alloy, but no appreciable difference is observed in as-cast and pre-solution treated ZA52Y2 specimens during the primary creep stage. They enter the secondary creep period at about 18 h. However, it still can be discerned in Fig.3(b) that pre-solution treated specimen experiences a slightly larger creep rate during the primary creep period. Difference between two curves becomes evident during the secondary creep period, and pre-solution treated specimen exhibits a relatively small creep rate during the secondary period. Through the comparison of creep behaviors of as-cast and pre-solution treated ZA52 and ZA52Y2 alloys, during the primary creep period, as-cast specimens exhibit a relatively small creep rate; while in the secondary creep period, pre-solution treated specimens exhibit relatively small creep rate.
Table 2 Minimum creep rate, primary creep strain and total creep strain of studied alloys(c—As-cast; s—Solution treated)
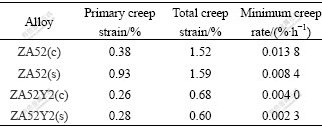
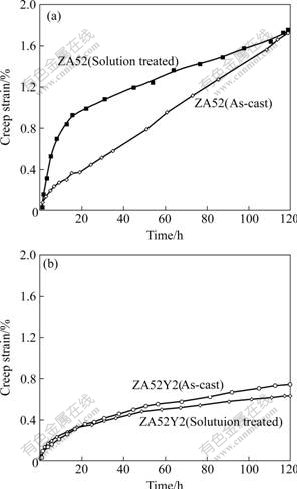
Fig.3 Comparison between creep curves of as-cast and solution-treated ZA52 (a) and ZA52Y2 (b) alloys
3.3 Crept microstructure and dynamic precipitation during creep deformation
The crept microstructures of as-cast ZA52 and ZA52Y2 alloy are shown in Figs.4(a) and (b), respectively. Some eutectic Mg-Zn lumps are found to depart from grain boundary in the crept microstructure of as-cast ZA52 specimen (by a distance of ΔS in Fig.4(a)), and SEM observation indicates that the eutectic Mg-Zn lumps in as-cast ZA52 and ZA52Y2 alloys exhibit a coarsening tendency (Figs.5(a) and 5(b)). EDAX analysis indicates the redistribution of alloying element occurs during the long period of creep, and the molar ratio of Al, Mg to Zn in Mg-Zn eutectic lumps is 24.21?51.17?24.13, deviating from its original ratio of 2.6?69.08?28.31. The morphology of intergranular Al11Y3 phase in as-cast ZA52Y2 alloy has changed during the creep deformation, and its branch structure disappears (Fig.6).
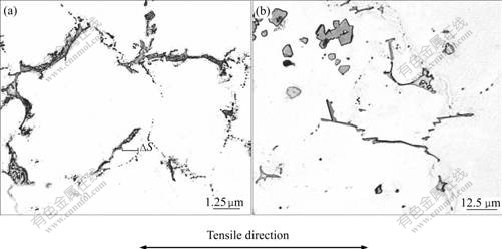
Fig.4 Crept microstructures of as-cast ZA52(a) and ZA52Y2(b) alloys (175 ℃, 50 MPa, 120 h) under OM
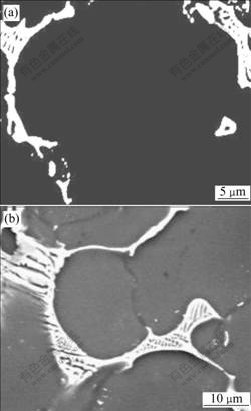
Fig.5 SEM images of Mg-Zn lumps in crept microstructure of ZA52(a) and ZA52Y2(b) alloys
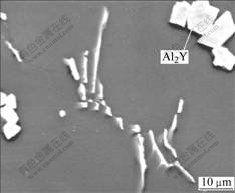
Fig.6 Branch-like phase distributing on grain boundary of crept ZA52Y2 alloy (SEM)
Low magnification TEM images of dynamic precipitates in the two pre-solution treated specimens are shown in Fig.7 (12 h, 175 ℃, 50 MPa). When the creep time reaches 12 h, tiny rods precipitate in the matrix, in a parallel way. However, some precipitation free zones are formed at one side of grain boundary in pre-solution treated alloys, which indicates that deformation bands exist at one side of grain boundary during the creep. Therefore, no dynamic precipitation occurs as a result of dense crystalline defects. Similar deformation bands are also found at some grain boundary areas of pre-solution treated ZA52Y2 alloys.
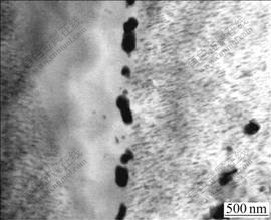
Fig.7 TEM image of grain boundary structure of pre-solution treated ZA52 during 12 h creep at 175 ℃ under load of 50 MPa
Bowing dislocation lines pinned by dynamic [0002]α rods are observed in two pre-solution treated specimens under TEM, as seen in Figs.8(a) and 8(b). Basal dislocation lines are observed in the creep specimens with the creep strains of 0.76% and 0.25%, respectively.
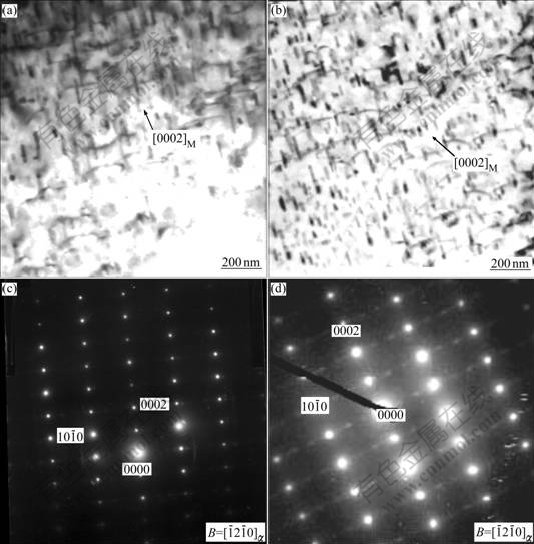
Fig.8 TEM morphologies of dynamic precipitate MgZn2 in pre-solution treated alloys: (a) ZA52 (with creep strain ε=0.67%); (b) ZA52Y2 ( with creep strain ε=0.67%); (c, d) Corresponding diffraction pattern of matrix
The SEAD pattern of rod-like dynamic precipitates is shown in Fig.9. It is indicated that the dynamically precipitated rods are transitional MgZn2 (η) precipitates, with a HCP crystalline structure. The calculation indicates the lattice parameters a≈0.523 3 nm, c≈0.856 6 nm[3], and the dynamic MgZn2 precipitates are found to obey the established orientation with α matrix: 
The θ-phase is proved to be the transitional precipitates parallel to basal plane.
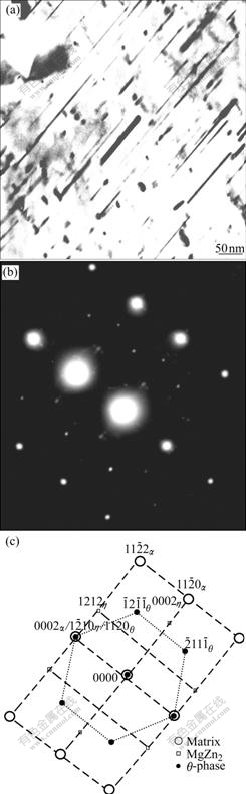
Fig.9 TEM morphology of dynamic precipitates(a) and composite diffraction pattern of MgZn2, θ precipitates and matrix with
(b, c)
4 Discussion
As the dominant alloying element of ZA52 alloy is Zn, Al element presents as a secondary element, and its content is less than 2.0%, far below its solubility at 335 ℃ according to Mg-Al-Zn ternary diagram[10]. Therefore, no g phase (Mg17Al12) is detected in the microstructure of as-cast ZA52 alloy. Mg51Zn20 eutectic lumps present as the dominant phase in grain boundary area. No other Al containing binary or ternary phase is detected in the microstructure of as-cast ZA52 alloy, and Al atoms exist in the form of solute in α matrix and eutectic lumps. The introduction of about 2.0% yttrium to ZA52 alloy leads to the formation of branch-structure Al11Y3 phase and polygonal Al2Y phase, mainly because of the thermodynamic priority of Al element over Mg and Zn elements when reacting with yttrium[11-13]. Branch-like Al11Y3 phase forms as a result of peritectic reaction between Al2Y particles and the liquid magnesium[12-14], in the course of the formation of α-dendrites. Therefore, Al2Y phase exhibits a higher thermodynamic stability over Al11Y3 phase at elevated temperatures. They often coexist in the microstructure of as-cast ZA52Y2 alloy, due to the incompleteness of peritectic reaction.
The formation of Al2Y and Al11Y3 phases depletes Al element in the melt, resulting in a lower Al content in ZA52Y2 alloy compared with ZA52 alloy, and this leads to the solution with increased Zn contents in α matrix of ZA52Y2 alloy, consequently, less Mg51Zn20 eutectic lumps in the microstructure of ZA52Y2 alloy.
Solution treatment leads to the dissolving of Mg51Zn20 eutectic phase into the α matrix of ZA52 and ZA52Y2 alloys. However, Al2Y particles and most branch-structure phases remain in the microstructure of ZA52Y2 alloy.
The creep behavior of an alloy is affected by many factors, among which the microstructure is the important one[15]. Two contradictory trends generally occur in the creep process: one is the softening process, and the other is the hardening process, such as atoms atmosphere, Orowan effect, and interweaving of dislocations. Therefore, the creep rate is determined by the interaction of above two contradictory trends. When the alloy is exposed to elevated temperatures, the grain boundary loses strength rapidly, therefore, creep deformation usually begins with boundary sliding. As for as-cast ZA52 alloy, its initial creep rate is relatively small compared with pre-solution treated specimens, mainly because of the strengthening effects of Mg-Zn eutectic lumps in the grain boundary area. As for ZA52Y2 alloy, the initial creep rate is mainly controlled by the branch-structure Al11Y3 phase at the grain boundary, and most branch-like Al11Y3 phase remains in the grain boundary area in pre-solution treated ZA52Y2 alloy. Therefore, only a slight distinction exists during their primary creep period.
Secondary phases in the grain boundary area play a very important role in retarding the creep deformation during the primary period, but their strengthening effects depend on their thermodynamic-stability and morphology. Many Mg-Zn eutectic lumps are observed deviating from grain boundary, and exhibit a coarsening tendency in the crept microstructure of as-cast alloys. Therefore, the strengthening effect of Mg-Zn lumps is weakened on the grain boundary with the proceeding of creep process. Compared with Mg-Zn eutectic lumps, the branch-structure Al11Y3 phase in ZA52Y2 alloy exhibits a strong strengthening effect on grain boundary, mainly due to its relatively high thermodynamic-stability and advantageous morphology. Therefore, both as-cast and solution treated ZA52Y2 specimen exhibits smaller primary creep strains than ZA52 alloy.
It seems that solution of Zn atoms in α matrix can hardly lead to an obvious strengthening effect during creep deformation, as pre-solution treated alloys exhibit larger creep rates during the primary period, mainly because of the large diffusion rate of Zn atoms at an elevated temperature. Yttrium atoms have a slow diffusion rate in ZA52Y2 alloy at an elevated temperature, but no yttrium element is detected by EDAX analysis in magnesium matrix before and after solution treatment, mainly because of the thermodynamic priority between Y and Al elements.
However, subsequent dynamic precipitation leads to a smaller minimum creep rate in two pre-solution treated alloys. Dynamic precipitation occurs in two pre-solution treated alloys at the end of primary period, and the majority of dynamic precipitates are identified as transitional MgZn2 phase. With the deepening of deformation, the piling up and the interweaving of dislocation lines lead to the hardening effect in the grain boundary area. As the deformation in the boundary area becomes more difficult, the creep strain is transmitted to the inner area of grains. Consequently, the strengthening role of evenly distributed MgZn2 precipitate is brought into play. MgZn2 rods act as an effective barrier to basal-plane dislocations, mainly because of its advantageous crystallographic orientation and relatively high thermo-stability.
The dynamic MgZn2 precipitates are observed to have a established orientation with matrix[16-18], with its
plane keeping a coherent relationship with
plane of α matrix. However, the effort of finding sheared MgZn2 rods under TEM is proved to be in vain. It is evident that dynamic MgZn2 rods still keep a fairly high thermodynamic stability and strength during creep deformation, so basal dislocation lines choose to bypass the MgZn2 rods rather than shear them under the experimental creep condition.
The deformation of magnesium alloys proceeds mainly in terms of sliding of a-dislocations in basal planes[19], however, TEM observation gives evidences of the operation of prismatic side system in the course of creep deformation, as seen in Fig.10. In order to lower down the energy of sliding, basal a-dislocations tend to move in terms of so-called extended dislocations. When the dynamic precipitation occurs, rod-like MgZn2 phase acts as an effective barrier to basal slide systems, consequently, the movement of basal dislocation system is impeded, leading to the pile-up of a-dislocations. In the meantime, the constriction of extended dislocations is facilitated by their pile-up in front of dynamic MgZn2 phase.
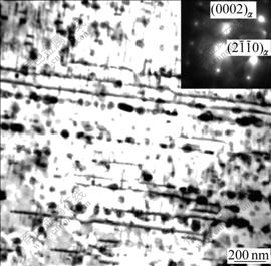
Fig.10 TEM image of dislocations on
prismatic plane in solution-treated ZA52 alloy during creep (at creep time of 24 h)
The critical P-N stress needed for the operation of slide systems on the basal and prismatic plane can be calculated by[15]
(1)
where σc is the critical stress required to operate the slide systems. ν is Poisson ratio, which can be regarded as 0.28 for pure magnesium. a is the interplanar spacing, 0.278 nm for
plan and 0.260 nm for basal planes. b is Burger’s vector. G is shear modulus, and can be calculated according to following equation[15]:
G =1.92×104-8.6T (2)
The relative difficulty for the operation of a-dislocations on prismatic and basal plane can be assessed in the following equation:
(3)
Therefore, the critical P-N stress needed for the operation of a-dislocations on prismatic
plane is about three times bigger than that on basal plane, which indicates a-dislocations can only be operated on prismatic
planes provided that the number of pinned dislocations on basal plane surpasses 4.
The calculation and analysis above indicate that the movement of basal slide system is impeded by the dynamically precipitated MgZn2 rods, and causes the cross-slip of basal a-dislocations to prismatic
plane. The relatively large critical P-N stress in prismatic
plane leads to smaller creep rates of solution treated alloys during the secondary period.
5 Conclusions
1) Intergranular intermetallic compounds Mg51Zn20 and Al11Y3 contribute to the smaller creep rates of as-cast ZA52 and ZA52Y2 alloys during the primary creep period. The strengthening effect of Mg51Zn20 is relatively weak due to its lower thermodynamic stability.
2) Dynamic MgZn2 phase precipitates in pre- solution treated ZA52 and ZA52Y2 alloys at the end of the primary creep period.
3) The formation of dynamic MgZn2 precipitates causes the cross-slip of basal a-dislocations to the prismatic
plane, leading to the smaller creep rates of solution-treated ZA52 and ZA52Y2 alloys during the secondary creep period.
References
[1] MORDIKE B L, EBERT T. Magnesium: Properties—applications— potential [J]. Materials Science & Engineering A, 2001, 302: 37-45.
[2] BROWN R. Magnesium automotive meeting [J]. Light Met Age, 1992, 50: 18-20.
[3] MAGERS D. Global review of magnesium parts in automobiles [J]. Light Met Age, 1997, 55: 60-62.
[4] SUZUKI M, KIMURA T, KOIKE J, MARUYAMA K. Effects of zinc on creep behavior and deformation substructures of Mg-Y alloy [J]. Materials Science Forum, 2003, 419/422: 473-478.
[5] LUO A, PEKGULERYUZ M O. Review—Cast magnesium alloys for elevated temperature applications [J]. Journal of Materials Science, 1994, 29: 5295-5271.
[6] MORENO I P, NANDY T K, JONES J W. Microstructural characterization of a die-cast magnesium-rare earth alloy [J]. Scripta Materialia, 2001, 45: 1423-1429.
[7] MORENO I P, NANDY T K, JONES J W. Microstructural stability and creep of rare-earth containing magnesium alloys [J]. Scripta Materialia, 2003, 45: 1029-1034.
[8] POWELL B R, REZHETS V, BALOGH M P, WALDO R A. Magnesium technology 2001[M]. New York: TMS, 2001: 175-180.
[9] ZHANG Zhan, COUTURE A. An investigation of the properties of Mg-Zn-Al alloys [J]. Scripta Materialia, 1998, 39: 45-53.
[10] VILLARS P, ALAN P. Handbook of ternary alloy phase diagrams [M]. OH: Materials Park, 1995: 3951-3951.
[11] LEE S, LEE S H, KIM D H. Effect of Y, Sr, and Nd additions on the microstructure and microfracture mechanism of squeeze-cast AZ91-X magnesium alloys [J]. Metall Mater Trans A, 1998, 29A: 1221-1235.
[12] WEI L Y, DUNLOP G L. The solidification behavior of Mg-Al-rare earth alloys [J]. Journal of Alloys and Compounds, 1996, 232: 264-268.
[13] KIM S H, KIM D H, KIM N J. Structure and properties of rapidly solidified Mg-Al-Zn-Nd alloys [J]. Materials Science & Engineering A, 1997, 226/228: 1030-1034.
[14] ZOU Hong-hui, ZENG Xiao-qin, ZHAI Chun-quan, DING Wen-jiang. The effects of yttrium element on the microstructure and mechanical properties of Mg-5%Zn-2%Al alloy [J]. Materials Science & Engineering A, 2005, 402: 142-148.
[15] REGEV M, ROSEN A, BAMBERGER M. Qualitative model for creep of AZ91D magnesium alloys [J]. Metal Mater Trans A, 2001, 32 (1): 1335-1345.
[16] STURKEY L, CLARK J B. Mechanism of age-hardening in the magnesium-zinc alloys [J]. Journal of the Institute of Metals, 1959, 88: 177-181.
[17] WEI L Y, DUNLOP G L, WESTENGEN H. Precipitation hardening of Mg-Zn and Mg-Zn-RE alloys [J]. Metal Mat Trans A, 1995, 26(1): 1705-1716.
[18] CLARK J B. Transmission electron microscopy study of age hardening in a Mg-5%Zn alloy [J]. Acta Metal, 1965, 13: 1281- 1289.
[19] NIE J F. Effects of precipitate shape and orientation on dispersion strengthening in magnesium alloys [J]. Scripta Materialia, 2003, 48: 1009-1015.
Corresponding author: ZOU Hong-hui; Tel: +86-10-82241289; Fax: +86-10-62055412; E-mail: hh_zou@eyou.com
(Edited by YANG Bing)