- Abstract:
- 1 Introduction▲
- 2 Governing equations, si...▲
- 3 Results and discussion ▲
- 4 Conclusions▲
- References
- Figure
- Fig.1 Mean streamwise velocity profiles as function of distance from wall
- Fig.2 Axial vortex rotation time scale, 1/ωx+,rms, as a function of distance from wall
- Fig.3 Convection velocities for vorticity perturbations in flow at different extents of DR
- Fig.4 Average time scales and ratio (=vortex life duration/ vortex rotation time) as function of extent of DR
J. Cent. South Univ. Technol. (2008) 15(s1): 275-279
DOI: 10.1007/s11771-008-362-7
Influence of rheological parameters in all drag reduction regimes of turbulent channel flow with polymer additives
LI Chang-feng(李昌烽), ZHAO Zuo-guang(赵作广), WU Gui-fen(吴桂芬), FENG Xiao-dong(冯晓东)
(School of Energy and Power Engineering, Jiangsu University, Zhenjiang 212013, China)
Abstract:
The influence of rheological parameters on vortex dynamics and the extent of drag reduction (DR) were deciphered via extensively analyzing the hi-fidelity direct numerical simulation results of the turbulent channel flow with polymer solutions. It has been observed that in all drag reduction regimes from the onset of DR to maximum drag reduction (MDR) limit, the Deborah number is defined as the product of an effective Weissenberg number, and the root mean square streamwise vorticity fluctuation remains O(1) in the near wall region. The ratio of the average lifetime of axial vortices to the vortex rotating duration decreases with increasing DR, and MDR is achieved when these time scales become nearly equal. Based on these observations a simple framework is proposed adequately to describe the influence of polymer additives on the extent of DR from onset to MDR as well as the universality of the MDR in flow systems with polymer additives.
Key words:
drag reduction; dilute polymeric solutions; turbulent channel flows; FENE-P model;
1 Introduction
It is well known that the addition of a small amount of soluble high molecular weight polymers to wall bounded turbulent flows can lead to dramatic drag reduction (DR). Specifically, it has been experimentally observed that polymer concentrations of O(100)×10-6 are sufficient to reduce drag up to 80%. Comprehensive reviews of the literature in this area are given in Refs. [1-4]. Two important aspects of this phenomenon are the existence of a threshold for the onset of drag reduction, as well an upper bound commonly referred to as the maximum drag reduction (MDR) limit. In addition, this upper bound of approximately 80% is insensitive to the chemical detail, concentration, molecular mass of the polymer over a broad range.
It is also well known that in wall bounded Newtonian turbulent flows, coherent structures play a central role in near-wall turbulence both in terms of turbulence production and eddy dissipation (i.e. a self-sustained dynamics of the mechanism)[5-7]. A number of experimental and computational studies have examined the modification of coherent structures and vortices responsible for the production of Reynolds stress by polymer additives[8-18]. Based on the observations two main mechanisms have been proposed, ‘viscous’ by LUMLEY[1-2], METZNER and coworkers[8] and ‘elastic’ by De GENNES[19]. Recent direct numerical simulation (DNS) results have provided support for the ‘viscous’ mechanism, namely, due to the strong bi-axial extensional bursts polymers undergoing a significant stretching resulting in the enhanced elongational viscosity of the solution and hence drag reduction[20].
Despite the large number of studies that have examined the influence of polymer additives on turbulent dynamics, relatively little is known about vortex dynamics and polymer micro-structure coupling. The goal of the present study is to elucidate the vortex dynamics in a turbulent channel flow of dilute polymer solutions with the aim of establishing the interaction between polymer chain and vortex dynamics. This will in turn be used to shed light on the mechanism of polymer turbulent drag reduction.
2 Governing equations, simulation technique and parameter selection
In the past decade it has been shown that continuum-level simulations based on elastic dumbbell models such as the FENE-P model (finitely extensible nonlinear elastic dumbbell model with the Peterlin closure) can qualitatively predict DR and the accompanying flow modification[15-17]. For the channel Poiseuille flow considered in this study, the flow dynamics and polymer stress fields can be fully characterized by four dimensionless groups, namely, Reτ, Weτ, β??, and L. The friction Reynolds and Weissenberg numbers, Reτ0 = hUτ/v0, Weτ0 = Uτ/v0, where h is the half-width of the channel; Uτ is the friction velocity; v0 is the zero shear rate kinematic viscosity, and λ is the polymer relaxation time. β=hs/h0, the ratio of the solvent (hs) to the total solution zero shear-rate viscosity (h0), is inversely related to the polymer concentration. L is the maximum chain extensibility. The polymeric stress tensor is related to the departure of the conformation tensor
from its equilibrium unit isotropic tensor state
, as
. The numerical
method used for solving the governing equations, rheological parameter sets and turbulence statistics to the extent of DR have been shown in detail in our previous paper[21]. It should be mentioned that present simulation results are gained from the streamwise computational domains (about 5 000 and 10 000 wall units), appropriately two or four times longer than that commonly applied for the Newtonian flow, which have been confirmed sufficiently long to accurately capture the dynamics of the flow in the HDR/MDR regime.
3 Results and discussion
Fig.1 shows the typical mean streamwise velocity profiles as a function of the distance from the wall for the simulation cases at Reτ? = 395. It is obvious that two DR regimes can be distinguished with different feathers as low drag reduction (LDR, 0<DR<30%-40%) and high drag reduction (HDR, DR>30%-40%)[13-14]. In the LDR regime, the logarithmic profile is shifted upwards parallel to that of the Newtonian flow. While in the HDR regime, the slope of the mean velocity is augmented gradually with the increasing DR levels. Finally, for the 74% DR case Virk’s MDR asymptote (U + =11.7y +-17) is approximately observed. The turbulence statistics has also been observed to be different between LDR and HDR regimes[13-14, 21].
Fig.1 Mean streamwise velocity profiles as function of distance from wall
The typical vorticity fluctuations ?at various DR levels up to MDR have been illustrated in Ref.[21]. The intensity of the streamwise vorticity fluctuations monotonically decreases with increasing DR, the streamwise vortex average sizes are increased while the average locations have been shifted to the center of the channel. It is well known that the streamwise vortices play an important role in mediating the mass/momentum transfer between the near wall region and turbulent core by ejecting low speed fluid from the near wall region to the core (ejection/upwash) as well as by bringing high speed fluid from the core towards the wall (sweep/downwash). Hence, it is of interest to examine the reciprocals of the streamwise vorticity fluctuations as it is a measure of the time scale associated with the vortex rotation speed. Of particular interest is the relationship between this time scale and the polymer time scale in wall units, i.e. Weτ. As shown in Fig.2, this time scale increases with increasing drag reduction. It can also be seen that in the viscous sublayer and buffer layer for Newtonian flows, this time scale is in the range of 5 to 10 in wall units. If drag reduction is manifested when these time scales at small y+ become equivalent (i.e., the dimensionless time scale for upwash and downwash become similar to Weτ), then one would expect that the onset of DR occurs in 5≤Weτ<0. Indeed, this is consistent with the findings for the onset of drag reduction at Weτ=6.25 (i.e. Deborah number, De= Weτ~O(1)[1-2, 8]). This is consistent with the ‘time criterion’ about the onset of drag reduction in the ‘viscous’ theory of polymer induced drag reduction. The time criterion indicates that drag reduction occurs when the polymer relaxation time is longer than the flow time scale of the near-wall turbulence, i.e. the friction Weissenberg Weτ exceeds an O(1) value.
This analysis clearly suggests that the near wall vortex time scale plays a significant role in determining the onset of DR. In fact, in addition to the onset of DR
Fig.2 Axial vortex rotation time scale, 1/ωx+,rms, as a function of distance from wall
we have observed that this time scale plays a significant role in all DR regimes. In the present FENE-P model, the extent to which the polymer chains have been extended should be considered rather than the maximum chain extensibility L. Thus an effective Weissenberg number Weτeff = Weτ[1-trace()/L2], is more accurate than We? to represent the polymer time scale during the interplay process between polymer chain and flow dynamics. The factor of [1-trace(
)/L2] can be found in the expression of the polymeric stress tensor. The values of Weτeff and 1/ωx+, rms in the near wall region are listed in Table 1. It is well known that the polymer chains are mostly stretched in the close wall region, and as the chain length is enhanced, trace(
) monotonically increases at a fixed Weτ, however the percentage of the average chain length, trace(
)/L2, is decreased[21].
Table 1 Comparison of Weτeff and 1/ωx+,rms in near wall region
It can be seen that from the onset of DR to MDR asymptote, the value of Weτeff is increased from the magnitude of 5-10 to about 60-70, while the production of Weτeffωx,+rms is kept of the order O(1) in the near wall region. This suggests that as elastic forces are enhanced they lead to stabilization of near wall axial vortices, resulting in much longer and slower rotating vortices. Furthermore, these findings underline the fact that there exists an intricate balance between elastic forces and average rotation speed of the near-wall axial vortices that determines upwash and downwash events and Reynolds stress production.
To shed light on the exact mechanism by which polymer induced drag reduction occurs, one needs to examine the various time scales of the flow. As mentioned above, we know that there exists an intricate balance between elastic forces and average rotation speed of the near-wall axial vortices, expressing Weτeffωx+rms ~O(1) in the time scale relationship. For a self-sustaining mechanism vortex life duration is another important time scale for the average vortex dynamics. The average vortex life duration can be derived from the average vortex length divided by the vortex convection velocity.
Fig.3 shows the convection velocities for the vorticity perturbations in the flow at different extent of DR. The convection velocity is determined by Dxmax/Dt, where Dxmax is the maximum for a given Dt from the time-space correlation[22]. It is now widely accepted that for the Newtonian flow the axial vorticity perturbations propagate at a constant speed about 10Ut within y+<10. It can be seen that with increasing DR, the same feature still exists, while the convection velocities are increased and the constant convection velocity regions are thickened as well. The intersection points between the convection velocity curves and the mean velocity profiles can be roughly regarded as the center of those energetic axial vortices. This implies that in the drag reduction flows the energetic axial vortices propagate at higher speeds with significantly increased eddy sizes. It can be found that at MDR the average axial vortices convect more than 3 times faster than Newtonian ones, and the average eddy diameter is at least 3 times as large as in Newtonian flows.
Two time scales are shown in Fig.4 for the vortex
Fig.3 Convection velocities for vorticity perturbations in flow at different extents of DR
Fig.4 Average time scales and ratio (=vortex life duration/ vortex rotation time) as function of extent of DR
life duration and vortex rotation period. Since the vortex length increase is larger than the vortex convection velocity increase, the average vortex life duration is increased with the extent of DR. The ratio of the vortex life duration to the vortex rotation time scale is also plotted in Fig.4 labeled in the right coordinate. It can be seen that although the average lifetime of axial vortices increases with increasing DR while their rotation speed decreases, the rate of decrease in the rotation speed exceeds the enhancement in lifetime of axial vortices as a function of DR. The ratio is more than 3 for Newtonian flow and down close to 1 as MDR approaching. Our results for Newtonian flow are in consistent with the fact that there are about 3 averaged ejection events during one bursting[11].
If further DR can be achieved higher than MDR, this time ratio needs to be less than 1. Then a full vortex rotation process cannot be completed within a vortex life duration, and the balance between elastic forces and vortex rotation will be broken. Thus the flow will go back to the laminar state where no polymer stretching. However, this laminar state cannot remain so long, and the flow will return to the turbulent state by any instability of infinitesimal perturbations. Then the polymer chains are stretched in turbulent flows, and the interplay between vortex dynamics and polymer chains will adjust themselves to satisfy the time criterion Weeffωx,+rms ~O(1), making the time ratio decreasing and decreasing. Upon the time ratio just less than 1, the above process repeats itself, showing that the MDR limit on average cannot be exceeded. This process is in close correspondence with the experimental remark by VIRK[3] that close to the MDR asymptote the flow appears laminar and shows very high intermittent[21]. This process is also showing the universal feature of MDR limit, since it is the vortex life duration and the vortex rotation time scale that become nearly equal as the MDR approached, nothing to do with polymer species, molecular mass, or the polymer solvent pair.
It is gratifying to discover that our simple framework is capable of incorporating several recently proposed mechanisms of drag reduction by polymer additives, which has been discussed in our recent papers[23-24].
4 Conclusions
The effect of polymer additives on vortex dynamics and the extent of DR has been deciphered via extensively analyzing the hi-fidelity DNS results of polymer induced drag reduction in turbulent channel flows up to the MDR limit. It has been observed that from the onset of DR to MDR, the Deborah number defined as the product of an effective Weissenberg number and the rms streamwise vorticity fluctuation remains O(1) in the near wall region. Moreover, it is shown that the average lifetime of axial vortices increases with increasing DR while their rotation speed decreases. However, the rate of decrease in the rotation speed exceeds the enhancement in lifetime of axial vortices as a function of DR. Hence, MDR is achieved when these time scales become nearly equal. This simple framework can adequately describe the influence of polymer additives on extent of DR from onset to MDR as well as the universality of the MDR in flow systems with polymer additives. We hope this framework can also give some clues for the drag reduction modeling and scaling in the HDR and MDR regimes based on the time criterion in combination with changes in the vortex size.
References
[1] Lumley J L. Drag reduction by additives [J]. Annu Rev Fluid Mech, 1969, 1: 367-384.
[2] Lumley J L. Drag reduction in turbulent flow by polymer additives [J]. Journal of Polymer Science, 1973, 7: 263-290.
[3] VIRK P S. Drag reduction fundamentals [J]. AIChE Journal, 1975, 21: 625-656.
[4] GRAHAM M D. Drag reduction in turbulent flow of polymer solutions[C]// Binding D M, Walters K eds. Rheology Reviews, British Society of Rheology, 2004: 143-170.
[5] KLINE S J, REYNOLDS W C, SCHRAUB F A, RUNSTADLER P W. The structure of turbulent boundary layers [J]. J Fluid Mech, 1967, 30: 741-773.
[6] KIM H T, KLINE S J, REYNOLDS W C. The production of turbulence near a smooth wall in a turbulent boundary layer [J]. J Fluid Mech, 1971, 50: 133-160.
[7] KIM J, MOIN P, MOSER P. Turbulence statistics in fully developed channel flow at low Reynolds numbers [J]. J Fluid Mech, 1987, 177: 133-166.
[8] SEYER F A, METZNER A B. Turbulence phenomena in drag reducing systems [J]. AIChE J, 1969, 15: 426-434.
[9] DONOHUE G L, TIEDERMAN W G, REISCHMA M M. Flow visualization in the near-wall region in a drag reducing flow [J]. J Fluid Mech, 1972, 56: 559-575.
[10] ACHIA B U, THOMPSON D W. Structure of the turbulent boundary layer in drag reducing pipe flow [J]. J Fluid Mech, 1977, 81: 439-464.
[11] TIEDERMAN W G, LUCHIK T S, BOGARD D G. Wall-layer structure and drag reduction [J]. J Fluid Mech, 1985, 156: 419-437.
[12] Den TOONDER J M J, HULSEN M A, KUIKEN G D C, NIUWSTADT F T M. Drag reduction by polymers in a turbulent pipe flow: Numerical and laboratory experiments [J]. J Fluid Mech, 1997, 337: 193-231.
[13] WARHOLIC M D, MASSAH H, HANRATTY T J. Influence of drag reducing polymers on turbulence: Effects of Reynolds number, concentration and mixing [J]. Exps Fluids, 1999, 27: 461-472.
[14] PTASINSKI P K, BOERSMA B J, NIUWSTADT FTM, HULSEN M A, Van Den BRULE BHAA, HUNT JCR. Turbulent channel flow near maximum drag reduction: simulations, experiments and mechanisms [J]. J Fluid Mech, 2003, 490: 251-291.
[15] Sureshkumar R, BERIS A N, HANDLER R A. Direct numerical simulation of turbulent channel flow of a polymer solution [J]. Phys Fluids, 1997, 9: 743-755.
[16] De ANGELIS E, CASCIOLA C M, PIVA R. DNS of wall turbulence: Dilute polymers and self-sustaining mechanisms [J]. Comp Fluids, 2002, 31: 495-507.
[17] HOUSIADAS K D, BERIS A N. Polymer-induced drag reduction: Effects of the variations in elasticity and inertia in turbulent viscoelastic channel flow [J]. Phys Fluids, 2003, 15: 2369-2384.
[18] MIN T, YOO J, CHOI H, JOSEPH D D. Drag reduction by polymer additives in a turbulent channel flow [J]. J Fluid Mech, 2003, 486: 213-238.
[19] De GENNES P G. Towards a scaling theory of drag reduction [J]. Physica A, 1986, 140: 9-25.
[20] DUBIEF Y, WHITE C M, TERRAPON V E, SHAQFEH ESG, MOIN P, LELE S K. On the coherent drag reducing and turbulence enhancing behavior of polymers in wall flows [J]. J Fluid Mech, 2004, 514: 271-280.
[21] LI C F, Sureshkumar R, Khomami B. Influence of rheological parameters on polymer induced turbulent drag reduction [J]. J Non-Newton Fluid Mech, 2006, 140: 23-40
[22] KIM J, HUSSAIN F. Propagation velocity of perturbations in turbulent channel flow [J]. Phys Fluids, 2003, 5: 695-706.
[23] Kim K, Li C F, Sureshkumar R, Balachandar S, Adrian R J. Effects of the polymer stresses on eddy structures in drag-reduced turbulent channel flow [J]. J Fluid Mech, 2007, 584: 281-299.
[24] LI C F, Sureshkumar R, Khomami B. Polymer induced drag reduction: The interplay between vortex dynamics and drag reduction[J]. Phys Rev Lett , 2008 (submitted)
(Edited by HE Xue-feng)
Foundation item: Project (10672069) supported by the National Natural Science Foundation of China
Received date: 2008-06-25; Accepted date: 2008-08-05
Corresponding author: LI Chang-feng, Professor , Ph.D.; Tel: +86-13775378215; E-mail: cfli@ujs.edu.cn
- Influence of rheological parameters in all drag reduction regimes of turbulent channel flow with polymer additives
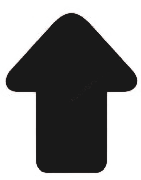