- Abstract:
- 1 Introduction▲
- 2 Experimental▲
- 3 Results and discus...▲
- 4 Conclusion▲
- References
- Figure
- Fig. 1 XRD patterns of graphite and GO
- Fig. 2 Raman spectra (a) and FTIR spectra (b) of graphite and GO
- Fig. 3 XPS survey scan (a) and high resolution C1s XPS spectrum (b) of GO
- Fig. 4 SEM micrographs (a, b) of GO at different magnifications and TEM images (c, d) of GO
- Fig. 5 Effect of volume fraction of GO on dielectric permittivity and dielectric loss at 103 Hz (a) and best fit of dielectric permittivity to Eq. (1) (b)
- Fig. 6 TGA-DSC curve of GO sample
- Fig. 7 SEM images of fractured surfaces:
- Fig. 8 Dependence of dielectric permittivity (a) and dielectric loss (b) of GO–PI films on frequency
J. Cent. South Univ. (2016) 23: 2747-2753
DOI: 10.1007/s11771-016-3336-1
Enhanced dielectric permittivity of graphite oxide/polyimide composite films
LI Jun(李俊), WANG Ya(王亚), LI Heng-feng(李衡峰)
School of Materials Science and Engineering, Central South University, Changsha 410083, China
Central South University Press and Springer-Verlag Berlin Heidelberg 2016
Abstract:
Graphite oxide (GO) was prepared by the pressurized oxidation method and incorporated into polyimide (PI) matrix to fabricate high-k composite films by in-situ polymerization and subsequent thermal treatment. The results show that the as-prepared GO had good dispersion and compatibility in PI matrix due to the introduction of abundant oxygen-containing functional groups during the oxidation. The residual graphitic domains and the thermal treatment induced reduction of GO further enhanced the dielectric permittivity of the resulting GO–PI composites. The dielectric permittivity of the GO–PI composites exhibited a typical percolation behavior with a percolation threshold of 0.0347 of volume ratio and a critical exponent of 0.837. Near the percolation threshold, the dielectric permittivity of the GO–PI composite increased to 108 at 102 Hz and was 26 times that of the pure PI.
Key words:
graphite oxide; pressurized oxidation; polyimide; dielectric permittivity; percolation;
1 Introduction
The development in microelectronic technologies has been raising higher claims on electronic packaging materials, where traditional ceramic-based materials cannot meet such requirements as microminiaturization/ light-weight trend of electronic devices and service on the organic substrates, owing to their high processing temperature and poor adhesive properties. Polymers possess many advantages including low cost, easy processing and great design flexibility, which are likely to meet those requirements [1-2] Among them, polyimide (PI) is an ideal polymer matrix material for electronic packaging due to its excellent electrical, mechanical and thermal performance, facile large-area film casting, and fine adhesive properties with organic substrates. Though PI is well-known as insulating material in electronics, PI-based high-k materials have also attracted considerable attention, especially for applications at high temperature where other polymers might fail. In recent years, incorporating conductive fillers into PI matrix has been a promising strategy for fabricating high dielectric permittivity PI-based composites. By introducing conductive fillers such as metals, carbon fiber, and carbon nanotubes, PI composites have shown distinctly enhanced dielectric permittivity when forming a percolative network in the host polymer [3-7].
Graphene is a newly emerging material with a unique 2D-layered structure and is widely used as a filler of multifunctional polymer composites with versatile applications [8-10]. It is well accepted that graphene is an ideal filler for enhancing the dielectric permittivity of polymer composites in respect of its largest aspect ratio and superior conductivity, but the poor dispersibility makes it easy to aggregate and form conductive path in the polymer matrix, thus damaging the dielectric performances of materials [11-14]. In contrast, graphite oxide (GO), a low-cost precursor material of graphene, has good dispersion and compatibility in many organic solvents and polymers, attributing to the existence of abundant oxygen-containing functional groups on its surface [10]. Furthermore, it was reported that the oxygen-containing functional groups on GO could be easily reduced by many reducing agents, such as hydrazine, alkali, vitamin C, hydrohalic acid, and N,N-dimethylformamide (DMF), transforming GO into reduced graphene oxide (rGO) with reconstructed conjugated planar structure and enhanced electrical conductivity [14-19].
In consideration of the good dispersion and adjustable electrical conductivity of GO, we incorporated GO into PI matrix by in-situ polymerization and subsequent thermal treatment to fabricate high-k composite films. To investigate the dielectric properties of the composites, a series of tests were performed on the as-prepared films, and the percolation theory was used to explain the dielectric behavior.
2 Experimental
2.1 Materials
3,3,4,4-benzophenonetetracarboxylic dianhydride (BTDA) (Beijing Multi Technology Co., Ltd., China) and 4,4-diaminodiphenyl ether (ODA) (Shanghai Jiachen Chemical Co., Ltd., China) were used as-received. Graphite flakes with high purity (300 mesh) were supplied by Nanjing XFNANO Materials Tech Co., Ltd. China. Sulfuric acid (H2SO4), potassium permanganate (KMnO4), hydrogen peroxide (H2O2), hydrochloric acid (HCl), and DMF were purchased from Sinopharm Chemical Reagent Co., Ltd. China and used without further purification.
2.2 Preparation of GO
GO was synthesized by the pressurized oxidation method following the procedure in Ref. [20]. Typically, graphite flakes, KMnO4, and H2SO4 were precooled to 0 °C. The cooled graphite flakes (0.6 g) were then placed in a reactor, followed by the addition of KMnO4 (3 g) and H2SO4 (30 mL). The reactor was sealed off immediately and placed in a stainless steel autoclave at 0 °C for another 1 h for complete mixing of the materials and then transferred to an oven at 80 °C for 2 h to complete the reaction. The reaction mixture was cooled to room temperature, and the obtained mud was diluted with 200 mL deionized water. Under vigorous stirring, 2.5 mL of H2O2 (30%) was gradually added into the suspension. The suspension was filtered and washed with HCl (10%) and deionized water until the pH reached 7. The gelatinous GO thus obtained was dried at 40 °C under vacuum for 48 h to obtain GO powder.
2.3 Preparation of GO–PI composite films
In a typical operation, GO (0.0575 g) was dispersed in 12 mL of DMF under sonication for 0.5 h in a 150 mL three-necked flask. ODA (0.7660 g) was added in the dispersion, followed by the gradual addition of BTDA (1.2640 g). The resulting mixture was stirred under argon for 24 h to obtain viscous GO–polyamic acid (PAA) solution. The film specimens were fabricated using the solution casting method. The films on glass substrates were placed in an oven overnight at 60 °C to evaporate most solvent and then heated at 80, 120 and 140 °C for 1 h, and 180, 220, 250 °C for 2 h, to completely remove the volatile materials and achieve thermal imidization. The GO–PI specimens containing 0.5%, 1%, 1.5%, 2%, 2.5%, 3%, 4%, 5% and 6% of GO were fabricated by the same steps. For comparison, the pure PI film was fabricated in the same manner. The thicknesses of the as-prepared films were in the range of 50–100 μm.
2.4 Characterization
Fourier transform infrared spectroscopy (FTIR, Nicolet 6700 spectrometer), X-ray diffraction (XRD, Rigaku D/Max 2500), Raman spectroscopy (LabRAM HR-800), and X-ray photoelectron spectroscopy (XPS, ESCALAB, Thermo Fisher Scientific) were used to characterize the structure and composition of the GO samples. The morphologies of the GO were recorded by a field-emission scanning electron microscope (SEM, FEI Sirion 200) and a transmission electron microscope (TEM, FEI Tecnai G220). The morphologies of the fractured surface of the samples were studied by SEM. The thermal properties of the obtained GO samples were investigated by thermogravimetric analysis-differential scanning calorimetry (TGA-DSC, NETZSCH DSC 200 F3) under argon from room temperature to 800 °C at a heating rate of 10 °C/min. The dielectric properties of the GO–PI samples were measured using an impedance analyzer (6500B, Wayne Kerr Electronics) over the frequency range of 102–106 Hz at room temperature.
3 Results and discussion
3.1 Characterization of GO
Figure 1 shows the XRD patterns of GO and the pristine graphite. The XRD pattern of graphite shows a sharp diffraction peak from (002) at 2θ value of ~26°, corresponding to an interlayer spacing of 0.34 nm. After the pressurized oxidation process, the diffraction peak at 26° obviously shifted to a 2θ value of ~10°, and the interlayer spacing increased to 0.89 nm. These changes indicate that the oxygen-containing functional groups were incorporated into the interlayer of the GO during the chemical oxidation process.
The Raman spectra reflect the structural features of graphite and GO, as shown in Fig. 2(a). Graphite shows the G and 2D bands at 1580 and 2700 cm-1, respectively. In addition, a weak D band at 1350 cm-1 in the Raman spectrum of graphite is attributed to the presence of tiny structural defects in graphite. In the case of GO, the intensity of D band, representing the structural defects, increased significantly. Besides, the D and G bands in GO show a blue shift to 1374 and 1623 cm-1, respectively, with the increased peak FWHMs (full width at half maximum), revealing the more defects and higher level of disorder in GO caused by the oxidation. Furthermore, the 2D band in the GO splits into two peaks with blunt shape, indicating that the obtained GO had a multilayer structure [21].
Fig. 1 XRD patterns of graphite and GO
Fig. 2 Raman spectra (a) and FTIR spectra (b) of graphite and GO
In Fig. 2(b), the FTIR spectra indicate that various carbon-oxygen functional groups are grafted onto GO: C—O of the alkoxy group (1052 cm-1), C—O of the carboxyl group (1232 cm-1), C—OH (1400 cm-1), and C=O of the carboxyl, and carbonyl (1726 cm-1). The peak at 1600 cm-1 indicates the presence of unoxidized graphitic domains in GO [22].
XPS offers direct information about the surface elemental composition and functional groups of GO. The XPS survey scan of GO and the corresponding C1s spectrum are shown in Fig. 3. The XPS survey scan shows a significantly intense O 1s peak in GO, and the corresponding C-to-O atomic ratio is 2:1, confirming the successful oxidation process of graphite. The C 1s XPS spectrum of GO is split into four peaks and can be attributed to carbon atoms with different functional groups, including sp2 C=C (284.3 eV), sp3 C—C (284.9 eV), C—OH (285.6 eV), C—O—C (286.9 eV), C=O (288.3 eV), and O=C (O) (288.3 eV) groups. These data prove that the pressurized oxidization process is effective, and the graphite is transformed into GO with successful introduction of various oxygen- containing groups. Moreover, the presence of sp2 C=C (284.3 eV) signal suggests that the oxidization is not complete and is in agreement with the FTIR spectrum of GO [23].
Fig. 3 XPS survey scan (a) and high resolution C1s XPS spectrum (b) of GO
The morphologies of GO were investigated by SEM and TEM, as shown in Fig. 4. The lateral views of the obtained GO show a loose and vesicular structure, indicating that various oxygen-containing functional groups were intercalated between layers after oxidation treatment. In the TEM images, the obtained GO well dispersed in the DMF solution exhibits a typical smooth carpet-like structure with the planar size of several micrometers, and the wrinkled surface indicates the presence of carbon–oxygen functional groups on GO [24]. The unique 2D structure with high aspect ratios would enhance the contact area of GO within polymeric matrices, which is a crucial factor for improving the dielectric performance of GO-polymer composites.
Fig. 4 SEM micrographs (a, b) of GO at different magnifications and TEM images (c, d) of GO
3.2 Dielectric properties of GO–PI films
Figure 5(a) shows the effect of the GO volume fractions on the dielectric properties at 103 Hz and room temperature. Initially, the dielectric permittivity and dielectric loss increase slightly with increasing GO concentration when the doping amount is <0.02 volume fraction (3%). A sharp increase in the dielectric permittivity is observed as the fraction of the GO increases to 0.033 volume fraction (5%), while the dielectric loss remains a relatively low value. However, as the volume fraction increases further up to 0.04 volume faction (6%), the dielectric permittivity decreases dramatically and the dielectric loss further increases simultaneously. This can be explained by the percolation theory, which describes that the insulator-conductor transition phenomenon occurs in the insulating polymer matrix. According to the percolation theory, the electrical properties of two-phase random composites are dominated by the matrix polymer, when the amount of conductive additives is below the critical concentration, and the additives distribute randomly in polymer matrices. As the conductive fillers are added continuously, they tend to connect with each other and begin to affect the electrical properties of composites. Once the amount of conductive fillers increases to a critical concentration, the conductive fillers are in proximity to each other but insulated by thin layers of polymer matrix, forming many microcapacitors in the composite. These microcapacitors combine to form a “supercapacitor network”, thus significantly enhancing the dielectric permittivity of the composites. However, when the conductive fillers are added beyond the critical concentration, some conducting paths are formed, leading to current leakage, which decreases the dielectric permittivity and increases the dielectric loss [5, 14].
This enhancement in the dielectric permittivity ε can be further explained according to the power law as follows [25]:
(ffiller<>0) (1)
where ε0 is the dielectric permittivity of the PI matrix; ffiller is the volume fraction of the conductive filler within the polymer matrix; f0 is the critical volume fraction at the percolation threshold; and q is the critical exponent.
Figure 5(b) shows the fitted curve of Eq. (1) by applying the dielectric permittivity and GO volume fraction data (for fGO<>0), and the inset gives the linear fit of lg(f0-fGO) and lg(ε/ε0). The perfect linear fit relationship demonstrates that the enhancement in the dielectric permittivity of the GO–PI composite is induced by incorporating of the as-prepared GO powder into the PI matrix, and the best fit of the dielectric permittivity data provides f0=0.0347 and q=0.837. The calculated value of f0 is in good accordance with the experimental results.
Traditionally, GO is identified as an insulating material and is even used as a low-k filler into polymer matrices [24, 26-27]. Interestingly, it is a type of effective high-k filler in this study, and its percolation behavior in the polymer matrix is a perfect match of Eq. (1). This enhancement in the dielectric permittivity induced by incorporating GO into the PI matrix indicates that the as-prepared GO might be electrically conductive or converted into conducting materials by the heat processing. As confirmed by the FTIR and XPS analyses, the residual graphitic domains in GO demonstrate an incomplete oxidation process of graphite, attributing a certain degree of electrical conductivity to the GO materials. Furthermore, the reducing solvent DMF combined with the thermal treatment used in the GO–PI films processing could induce the reduction of GO sheets in the composites, resulting in further increased electrical conductivity [17]. In Fig. 6, the TGA-DSC curve of GO powder shows a sharp exothermic peak at ~200 °C accompanied with a dramatic weight loss, which is attributed to the decomposition of labile oxygen- containing functional groups presented on the GO surfaces. This feature indicates that the GO materials were reduced during the thermal treatment, affording an enhanced electrical conductivity.
Fig. 5 Effect of volume fraction of GO on dielectric permittivity and dielectric loss at 103 Hz (a) and best fit of dielectric permittivity to Eq. (1) (b)
Figure 7 shows the fractured images of the pure PI and GO–PI composite films with different amounts of GO. It is obvious that the fractured surface of pure PI is quite compact and smooth; while it is ridged and rough for the GO/PI composites, resulting from the 2D-layered geometry of GO. Meanwhile, GO fillers are homogeneously dispersed in the PI matrix due to the enhanced interaction between the oxygen functional groups introduced into GO layers and the polymeric matrix. It is also noted that as the GO loading is at a lower level, GO fillers are dispersed separately in the matrix (Figs. 7(b) and (c)), while as the GO loading increases to 5.0%, GO fillers seems to come into contact with each other and form a microcapacitor network, which might be responsible for the dramatically improved dielectric permittivity of the composites, as shown in Fig. 5(a).
Fig. 6 TGA-DSC curve of GO sample
Figure 8 shows the dependence of the dielectric permittivity and dielectric loss of the GO–PI composite films on the frequency with different GO contents. The dielectric permittivity and dielectric loss are found to be almost independent of the frequency and increase slightly with increasing GO loading when the additive amount is <3.0%, indicating that the PI matrix dominates the dielectric behavior. However, with further increase in the GO content, the dielectric permittivity and dielectric loss increase significantly in a lower frequency range (102-103 Hz) and exhibit dramatic decreases with the frequency. When the GO loading increases to 5% (0.033 volume fraction), which is near the percolation concentration, the maximum dielectric permittivity is 108 at 102 Hz, which is 26 times higher than that of the pure PI. The frequency dependence of dielectric properties is associated to the mechanism of polarization. The dielectric properties of the GO–PI films are dominated by the molecular polarization at a lower GO loading as the molecular polarization acquires short responding time. In other words, the dielectric response can keep pace with the frequency and thus the dielectric permittivity and dielectric loss are independent of frequency. With increasing GO loading, the strong interfacial polarization induced by the newborn interfaces between the GO and PI segments dominates the dielectric behavior of the GO–PI films. As the interfacial polarization requires longer time to respond, the dielectric permittivity and dielectric loss are significantly enhanced in the lower frequency range and decrease dramatically with the frequency [13-14].
Fig. 7 SEM images of fractured surfaces:
Fig. 8 Dependence of dielectric permittivity (a) and dielectric loss (b) of GO–PI films on frequency
4 Conclusion
High dielectric permittivity GO–PI composite films were prepared by in-situ polymerization and subsequent thermal treatment. The as-prepared GO showed good dispersion and compatibility in PI matrix due to the introduction of abundant oxygen-containing functional groups by the pressurized oxidation. The residual graphitic domains and the thermal treatment induced reduction of GO further enhanced the dielectric permittivity of the resulting GO–PI composites. The enhancement of the dielectric permittivity of GO–PI composites was explained by the percolation theory and microcapacitor model, providing a percolation threshold of 0.0347 in the volume fraction of GO. The maximum value of the dielectric permittivity for the GO–PI composites near the percolation concentration was 108 at 102 Hz, 26 times higher than that of the pure PI. The significantly improved dielectric permittivity induced by simply incorporating GO into PI might be a promising solution for fabricating high-k GO-PI composites.
References
[1] LU J, MOON K S, XU J, WONG C P. Synthesis and dielectric properties of novel high-K polymer composites containing in-situ formed silver nanoparticles for embedded capacitor applications [J]. Journal of Materials Chemistry, 2006, 16(16): 1543-1548.
[2] WANG Y, ZHOU X, CHEN Q, CHU B J, ZHANG Q M. Recent development of high energy density polymers for dielectric capacitors [J]. IEEE Transactions on Dielectrics and Electrical Insulation, 2010, 17(4): 1036-1042.
[3] WANG L, PIAO X, ZOU H, WANG Y, LI H. High dielectric, dynamic mechanical and thermal properties of polyimide composite film filled with carbon-coated silver nanowires [J]. Applied Physics A, 2015, 118(1): 243-248.
[4] HE G, ZHOU J, TAN K, LI H. Preparation, morphology and properties of acylchloride-grafted multiwall carbon nanotubes/ fluorinated polyimide composites [J]. Composites Science and Technology, 2011, 71(16): 1914-1920.
[5] DANG Z M, PENG B, XIE D, YAO S H, JIANG M J, BAI J. High dielectric permittivity silver/polyimide composite films with excellent thermal stability [J]. Applied Physics Letters, 2008, 92(11): 112910.
[6] XU J, WONG C P. Effect of the polymer matrices on the dielectric behavior of a percolative high-k polymer composite for embedded capacitor applications [J]. Journal of Electronic Materials, 2006, 35(5): 1087-1094.
[7] DANG Z M, WU J P, XU H P, YAO S H, JIANG M J, BAI J. Dielectric properties of upright carbon fiber filled poly(vinylidene fluoride) composite with low percolation threshold and weak temperature dependence [J]. Applied Physics Letters, 2007, 91(7): 072912.
[8] HA H W, CHOUDHURY A, KAMAL T, KIM D H, PARK S Y. Effect of chemical modification of graphene on mechanical, electrical, and thermal properties of polyimide/graphene nanocomposites [J]. ACS Applied Materials & Interfaces, 2012, 4(9): 4623-4630.
[9] HUANG T, XIN Y, LI T, NUTT S, SU C, CHEN H, LIU P, LAI Z. Modified graphene/polyimide nanocomposites: Reinforcing and tribological effects [J]. ACS Applied Materials & Interfaces, 2013, 5(11): 4878-4891.
[10] POTTS J R, DREYER D R, BIELAWSKI C W, RUOFF R S. Graphene-based polymer nanocomposites [J]. Polymer, 2011, 52(1): 5-25.
[11] LIU J, TIAN G, QI S, WU Z, WU D. Enhanced dielectric permittivity of a flexible three-phase polyimide–graphene–BaTiO3 composite material [J]. Materials Letters, 2014, 124: 117-119.
[12] TIAN G, SONG J, LIU J, QI S, WU D. Enhanced dielectric permittivity and thermal stability of graphene-polyimide nanohybrid films [J]. Soft Materials, 2014, 12(3): 290-296.
[13] LIU J, SONG J, QI S, TIAN G, WU D. Enhanced dielectric permittivity of fluorine polyimide matrix with embedded grapheme [J]. Physica Status Solidi – Rapid Research Letters, 2013, 7(8): 575- 578.
[14] FAN P, WANG L, YANG J, CHEN F, ZHONG M. Graphene/ poly(vinylidene fluoride) composites with high dielectric constant and low percolation threshold [J]. Nanotechnology, 2012, 23(36): 365702-365709.
[15] FAN X, PENG W, LI Y, LI X, WANG S, ZHANG G, ZHANG F. Deoxygenation of exfoliated graphite oxide under alkaline conditions: A green route to graphene preparation [J]. Advanced Materials, 2008, 20(23): 4490-4493.
[16] LIAO K H, MITTAL A, BOSE S, LEIGHTON C, MKHOYAN K A, MACOSKO C W. Aqueous only route toward graphene from graphite oxide [J]. ACS Nano, 2011, 5(2): 1253-1258.
[17] LIN Z, YAO Y, LI Z, LIU Y, LI Z, WONG C P. Solvent-assisted thermal reduction of graphite oxide [J]. The Journal of Physical Chemistry C, 2010, 114(35): 14819-14825.
[18] KOU L, LIU Z, HUANG T, ZHENG B, TIAN Z, DENG Z, GAO C. Wet-spun, porous, orientational graphene hydrogel films for high-performance supercapacitor electrodes [J]. Nanoscale, 2015, 7(9): 4080-4087.
[19] CHUA C K, PUMERA M. Chemical reduction of graphene oxide: A synthetic chemistry viewpoint [J]. Chemical Society Reviews, 2014, 43(1): 291-312.
[20] BAO C L, SONG L, XING W Y, YUAN B H, WILKIE C A, HUANG J L, GUO Y Q, HU Y. Preparation of graphene by pressurized oxidation and multiplex reduction and its polymer nanocomposites by masterbatch-based melt blending [J]. Journal of Materials Chemistry, 2012, 22(13): 6088-6096.
[21] ARSAT R, BREEDON M, SHAFIEI M, SPIZZIRI P G, GILJE S, KANER R B, KALANTAR-ZADEH K, WLODARSKI W. Graphene-like nano-sheets for surface acoustic wave gas sensor applications [J]. Chemical Physics Letters, 2009, 467(4/5/6): 344-347.
[22] DUTTA S, RAY C, SARKAR S, PRADHAN M, NEGISHI Y, PAL T. Silver nanoparticle decorated reduced graphene oxide (rGO) nanosheet: A platform for sers based low-level detection of uranyl ion [J]. ACS Applied Materials & Interfaces, 2013, 5(17): 8724-8732.
[23] DREYER D R, PARK S, BIELAWSKI C W, RUOFF R S. The chemistry of graphene oxide [J]. Chemical Society Reviews, 2010, 39(1): 228-240.
[24] LIAO W H, YANG S Y, HSIAO S T, WANG Y S, LI S M, TIEN H W, MA C C M, ZENG S J. A novel approach to prepare graphene oxide/soluble polyimide composite films with a low dielectric constant and high mechanical properties [J]. RSC Advances, 2014, 4(93): 51117-51125.
[25] NAN C W. Physics of inhomogeneous inorganic materials [J]. Progress in Materials Science, 1993, 37(1): 1-116.
[26] LIAO W H, YANG S Y, HSIAO S T, WANG Y S, LI S M, MA C C M, TIEN H W, ZENG S J. Effect of octa(aminophenyl) polyhedral oligomeric silsesquioxane functionalized graphene oxide on the mechanical and dielectric properties of polyimide composites [J]. ACS Applied Materials & Interfaces, 2014, 6(18): 15802-15812.
[27] WANG J Y, YANG S Y, HUANG Y L, TIEN H W, CHIN W K, MA C C M. Preparation and properties of graphene oxide/polyimide composite films with low dielectric constant and ultrahigh strength via in situpolymerization [J]. Journal of Materials Chemistry, 2011, 21(35): 13569-13575.
(Edited by YANG Hua)
Foundation item: Project(2013JSJJ002) supported by the Faculty Research Fund of Central South University, China
Received date: 2015-10-13; Accepted date: 2016-03-08
Corresponding author: LI Heng-feng, Professor, PhD; Tel: +86-731-88877793; Fax: +86-731-88877692; E-mail: lihf@csu.edu.cn
Abstract: Graphite oxide (GO) was prepared by the pressurized oxidation method and incorporated into polyimide (PI) matrix to fabricate high-k composite films by in-situ polymerization and subsequent thermal treatment. The results show that the as-prepared GO had good dispersion and compatibility in PI matrix due to the introduction of abundant oxygen-containing functional groups during the oxidation. The residual graphitic domains and the thermal treatment induced reduction of GO further enhanced the dielectric permittivity of the resulting GO–PI composites. The dielectric permittivity of the GO–PI composites exhibited a typical percolation behavior with a percolation threshold of 0.0347 of volume ratio and a critical exponent of 0.837. Near the percolation threshold, the dielectric permittivity of the GO–PI composite increased to 108 at 102 Hz and was 26 times that of the pure PI.
- Enhanced dielectric permittivity of graphite oxide/polyimide composite films
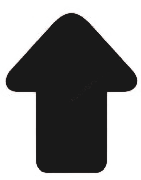