
Microstructure and mechanical properties of Mg-Cu-Y-Zn bulk metallic glass matrix composites prepared in low vacuum
WANG Jing-feng(王敬丰)1, WU Xia(吴 夏)1, PAN Fu-sheng(潘复生)1, TANG Ai-tao(汤爱涛)1,
DING Pei-dao(丁培道)1, LIU Ri-ping(刘日平)2
1. National Engineering Research Center for Magnesium Alloys, Chongqing University, Chongqing 400044, China;
2. State Key Laboratory of Metastable Materials Science and Technology, Yanshan University,
Qinhuangdao 066004, China
Received 12 June 2008; accepted 5 September 2008
Abstract: Mg-Cu-Y-Zn bulk metallic glasses (BMG) and in situ bulk metallic glass matrix composites were prepared by copper mold casting under the low vacuum with the argon atmosphere, and the raw materials used in preparing the Mg-Cu-Y-Zn alloys were commercially pure materials. The microstructures of the bulk samples were analyzed by X-ray diffractometer (XRD) and the thermal stability of samples was investigated by using a differential scanning calorimeter (DSC). The thermal stability of sample prepared with commercially pure raw materials is close to that of sample prepared with the high pure raw materials for the BMG Mg65+X(Cu0.66Y0.33)30-XZn5 (X=6). With the increase of Mg content, Mg-Cu-Y-Zn composites are prepared, in which Mg solid solution flakes and Y2O3 flakes are dispersed. In comparison with monolithic Mg-based BMG alloys, the composites exhibit significant improvement in mechanical properties, e.g. a compressive plastic strain about 7% and an ultimate strength of 1 170 MPa in Mg65+X(Cu0.66Y0.33)30-XZn5 (X=14). It is suggested that the enhancement of the mechanical properties of the composites can be attributed to the generation of multiple shear bands and the quantity of the Mg solid solution flakes.
Key words: bulk amorphous alloys; magnesium; commercially pure raw materials; microstructure; mechanical property
1 Introduction
Mg-based bulk metal glasses (BMG) exhibit great improvement in strength property and low density, particularly attractive for engineering applications[1-4]. But Mg-based bulk metal glasses have been found to be brittle at room temperature. They always fracture in the elastic regime without observable plastic deformation [5-7]. It has become one of the challenging problems for their engineering applications[8-10]. In the efforts to overcome the brittleness of BMG alloys, the preparation of BMG matrix composites by using in situ or ex situ methods has been proven to be an effective way. For example, the plastic strains to failure of Mg-based composites with ex situ TiB2 particles were found to reach the order of 2%-3%[11-12]. MA et al[13] synthesized Mg65Cu7.5Ni7.5Zn5Ag5Y10 BMG matrix in situ composites containing iron as secondary phase dispersions.
The macroscopic plasticity of the composite with 13% (volume fraction) of iron particles is about 1%, and the fracture strength is increased to 990 MPa. HUI et al[14] synthesized Mg-Cu-Y-Zn BMG matrix in situ composites containing Mg-based secondary phase with long-period order structure (LOS), the fracture strength is increased to 1 200 MPa, and the compressive plastic strain is increased to 18%.
But, it is noticeable that most Mg-based BMG matrix in situ composites are prepared under the high vacuum with the high pure raw materials[15-17], which increases the cost and is bad for their engineering applications[18-20]. So, in this work, the Mg-Cu-Y-Zn BMG matrix in situ composites were prepared by copper mold casting, under the low vacuum with the argon atmosphere, with commercially pure raw materials, and a way of using Mg-based BMG as engineering materials was provided.
2 Experimental
The nominal composition of the alloys studied in this work was Mg65+X(Cu0.66Y0.33)30-XZn5 (X=6, 10, 14) (molar fraction, %). The Cu-Y intermediate alloys were first arc melted under Ti-getter argon. The raw materials used in preparing Cu-Y intermediate were high pure metals: Cu (99.9%) and Y (99.9%). Then Mg-Cu-Y-Zn master ingots were prepared by high- frequency induction melting under low vacuum (1-5 Pa) with the argon atmosphere by using pre-alloyed Cu-Y intermediate alloy, and commercially pure Mg (99%) and Zn (99%) metals. Mg65+X(Cu0.66Y0.33)30-XZn5 (X=6, 10, 14) rod samples with 3 mm in diameter and 50 mm in length were fabricated by using a water-cooled copper mold casting method. The microstructures of the bulk samples were analyzed by X-ray diffraction (XRD) in a PHILIPS diffractometer (Cu Kα radiation) and TESCAN VEGAⅡ LMU scanning electron microscope (SEM).
Thermal stability of samples was investigated by using a differential scanning calorimeter (Perkin-Elmer DSC 7) at heating rate of 20 K/min in a flow of argon. The DSC system was calibrated for temperature and enthalpy by using zinc and indium standards, giving an accuracy of ±0.2 K and ±0.02 mW, respectively. The values of the glass transition temperature (Tg), the onset temperature (Tx) for crystallization, the melting temperatures Tm and Tl were determined from the DSC traces with an accuracy of ±1 K.
The value of fracture strength and compression strain of Mg65+X(Cu0.66Y0.33)30-XZn5 (X=6, 10, 14) was evaluated by compression test. The compression test samples were 3 mm in diameter and 5 mm in length, and the fracture morphologies of the samples were examined by SEM.
3 Results and discussion
Fig.1 shows the XRD patterns obtained from the transverse cross-section of the Mg65+X(Cu0.66Y0.33)30-XZn5
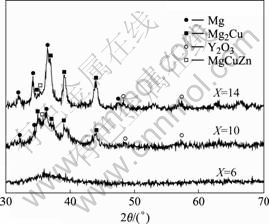
Fig.1 XRD patterns of Mg65+X(Cu0.66Y0.33)30-XZn5 (X=6, 10 and 14) samples
(X=6, 10 and 14). The XRD patterns for the alloy at X=6 consist only of broad diffraction peaks, indicating the formation of a single glass phase. When the concentration of magnesium is increased, the XRD patterns for the alloy at X=10 and 14 exhibit sharp peaks superimposed on a broad diffraction peak. The peaks are identified as Mg in major with Mg2Cu, Y2O3 and MgCuZn, indicating that the samples are the bulk metallic glass composites. As reported in Ref.[14], there exists a ternary reaction: L=α(Mg)+Mg2Cu+ (MgCu2+MgZn2) in the Cu-Mg-Zn ternary system at the composition of 78.6% Mg, 13.3% Cu and 8.1% Zn (molar fraction). It is deduced that the Mg, Mg2Cu and MgCuZn phases may be the products of solid-state transformation following the ternary eutectic reaction. In addition, the Y2O3 phase may be the product of oxidation transformation because these samples are prepared under low vacuum.
The SEM images of Mg65+X(Cu0.66Y0.33)30-XZn5 (X=6, 10 and 14) samples are shown in Fig.2. For the alloy at X=6 (Fig.2(a)), the sample exhibits featureless microstructure, indicating a fully glass structure. For the alloy at X=10 (Fig.2(b)), it is found that the black flake-
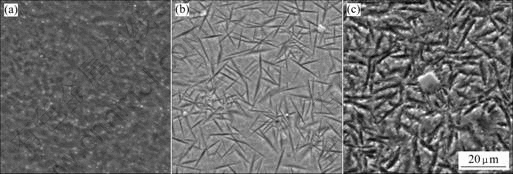
Fig.2 SEM images of Mg65+X(Cu0.66Y0.33)30-XZn5 samples: (a) X=6; (b) X=10; (c) X=14
shaped precipitates are uniformly distributed in the glass matrix. Some flakes intersect each other. With increasing concentration of magnesium, the amount of flake-shaped precipitates increases for the alloy at X=14 (Fig.2(c)). The flake-shaped precipitates are identified as Mg-based solid solution by EDS. In addition, some small white flakes which are found to be Y2O3 are detected in the SEM observation of the surface for the alloys at X=10 and 14. It is noticed that more Mg-based flake-shaped precipitates are observed around the Y2O3 flakes (melting point is 2 963 K), indicating that Y2O3 is the primary phase produced by solid-state transformation and can be heterogeneous nucleation center for the crystal grain.
The DSC traces obtained from the Mg65+X(Cu0.66- Y0.33)30-XZn5 (X=6, 10 and 14) samples with fully glass and BMG composite structure are shown in Fig.3. The glass transition temperature Tg, the onset temperature of the first exothermic reaction Tx, the super cooled liquid range ?Tx (?T=Tx-Tg), and the melting temperatures Tm and Tl are listed in Table 1. It is observed that DSC traces exhibit some exothermic peaks for all the alloys. But with increasing concentration of magnesium, the weight of the peaks decreases, indicating that the Mg65+X(Cu0.66- Y0.33)30-XZn5 (X=10 and 14) samples are BMG composites. With the increase in Mg concentration, ?T decreases from 36.4 K (X=6) to 28.64 K (X=10), which can be attributed to the crystalline phases in the alloys. In addition, the values of Tg, Tx and ?Tx (?Tx=Tx-Tg) of the Mg65+X(Cu0.66Y0.33)30-XZn5 (X=6) are 409.8, 446.2
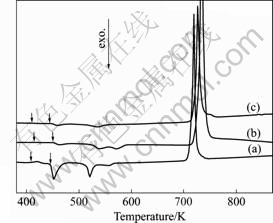
Fig.3 DSC traces of Mg65+X(Cu0.66Y0.33)30-XZn5 samples with heating rate of 20 K/min: (a) X=6; (b) X=10; (c) X=14
Table 1 Tg, Tx, ?Tx (?Tx=Tx-Tg), Tl and Tm of Mg65+X(Cu0.66Y0.33)30-XZn5(X=6, 10 and 14) samples
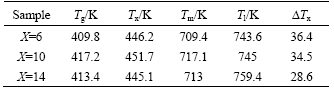
and 36.4 K in this work with commercially pure raw materials which are close to 409, 455 and 46 K for the alloy which was reported prepared with the high pure raw materials, indicating that the Mg-based BGM can be prepared under low vacuum, with commercially pure raw materials.
The compressive engineering stress—strain curves of as-cast Mg65+X(Cu0.66Y0.33)30-XZn5 (X=6, 10 and 14) samples with 3 mm-diameter and 5 mm-length are shown in Fig.4. Under quasistatic loading, the monolithic glass exhibits linear elastic behavior with an ultimate fracture strength δf=814 MPa, and no plastic deformation prior to failure is observed. With the increase of Mg concentration, significant improvement in mechanical properties is achieved due to the formation of Mg-based ?ake-shaped precipitates in the glass matrix. The alloy at X=10 exhibits fracture strength δf=1 104 MPa and plastic strain to failure εf=5.8%, For the alloy at X=14, the composite shows fracture strength δf=1 170 MPa and plastic strain to failure εf=7%. It is found that the increase of fracture strength and plastic strain of the composites attribute to Mg solid solution flakes.
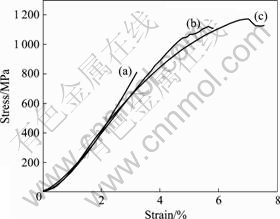
Fig.4 Room-temperature compressive stress—strain curves for Mg65+X(Cu0.66Y0.33)30-XZn5 samples: (a) X=6; (b) X=10; (c) X=14
The microscopic strength of typical Mg-based BMG alloys and the Mg BMG matrix composites are listed in Table 2. HV97 for the alloy at X=6, and HV168 and HV 185 for the alloy at X=10 and 14 indicate that the composites are more hard than the signal BMG.
Table 2 Microhardness of Mg65+X(Cu0.66Y0.33)30-XZn5 (X=6, 10 and 14) samples
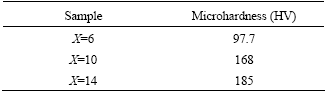
The fracture morphologies of the Mg65+X(Cu0.66Y0.33)30-XZn5 (X=6) monolithic metallic glass and Mg65+X(Cu0.66Y0.33)30-XZn5 (X=14) composites are shown in Fig.5. For the monolithic glass, as seen in Fig.5(a), the fracture surface is smooth and featureless. The shear bands are straight and propagate in an unimpeded fashion over rather large distances in the monolithic glass. The large smooth areas and the straight shear bands suggest that the alloy at X=6 is brittle.
For the Mg65+X(Cu0.66Y0.33)30-XZn5(X=14) composites, as seen in Fig.5(b) and 5(c), well-developed vein patterns
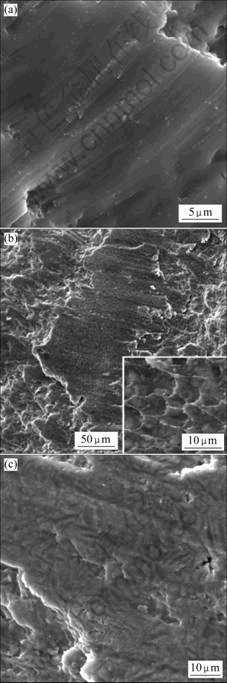
Fig.5 Fracture morphologies of Mg65+X(Cu0.66Y0.33)30-XZn5 samples: (a) X=6; (b); (c) X=14
and the flake-shaped precipitates are formed on the fracture surface. It is found that vein patterns are distributed uniformly over the whole areas (as shown in the inset of Fig.5(b)) due to the flake-shaped precipitates which hinder the propagation of shear bands and promote the formation of multiple shear bands. In addition, it is also noticeable that other different fracture zones which are smooth can be found. Uniformly distributed flakes are observed in the zones, but vein patterns do not. It is demonstrated that the fracture of the composite in this region is not only controlled by the deformation of the glass matrix but also controlled by the deformation of the Mg phase.
In conclusion, the Mg65+X(Cu0.66Y0.33)30-XZn5 (X=6) monolithic metallic glass is brittle as the result of the inhomogeneous shear bands. It fractures in the elastic regime without observable plastic deformation. For the Mg65+X(Cu0.66Y0.33)30-XZn5 (X=14) composites, the Mg-based flake-shaped precipitates which hinder the propagation of shear bands and promote the formation of multiple shear bands, and the regime which is fractured as the Mg are observed in this composites. Both of them improve the mechanical properties of the composites.
4 Conclusions
1) The Mg-Cu-Y-Zn bulk metallic glasses (BMG) and in situ bulk metallic glass matrix composites are prepared under the low vacuum (1-5 Pa) with the argon atmosphere, by using the commercially pure raw materials and a copper mold casting.
2) In comparison with monolithic Mg-based BMG alloys, the composites exhibit significant improvement in mechanical properties, e.g. a compressive plastic strain about 7% and an ultimate strength of 1 170 MPa in Mg65+X(Cu0.66Y0.33)30-XZn5 (X=14) with a dimension of 3 mm in diameter and 5 mm in length.
3) It is believed that the in situ bulk metallic glass matrix composites prepared under the low vacuum with the commercially pure raw materials provide a possibility of using Mg-based BMG as engineering materials.
Acknowledgements
This work was financially supported by the National Key Basic Research Program (973 Program) (No.2007CB613704), the National Natural Science Foundation of China (No. 50725413), Natural Science Foundation Project of CQ CSTC (Grant No. CSTS2006BB4199) and the State Key Laboratory of Metastable Materials Science and Technology, Yanshan University. The authors would like to thank Prof. LIU Lin at the Department of Materials Science and Engineering, Huazhong University of Science & Technology, China for help in samples preparation.
References
[1] LEE P Y, LIU W C, LIN C K, HUANG J C. Fabrication of Mg-Y-Cu bulk metallic glass by mechanical alloying and hot consolidation [J]. Mater Sci Eng A, 2007, 449/451: 1095-1098.
[2] XI X K, ZHAO D Q, PAN M X, WANG W H. Highly processable Mg65Cu25Tb10 bulk metallic glass [J]. Journal of Non-crystalline Solids, 2004, 344: 189-192.
[3] YUAN G Y, INOUE A. The effect of Ni substitution on the glass-forming ability and mechanical properties of Mg-Cu-Gd metallic glass alloys [J]. Journal of Alloys and Compounds, 2005, 387: 134-138.
[4] CHANG Y C, HUNG T H, CHEN H M, HUANG J C, NIEH T G, LEE C J. Viscous ?ow behavior and thermal properties of bulk amorphous Mg58Cu31Y11alloy [J]. Intermetallics, 2007, 15: 1303-1308.
[5] BARICCO M, CASTELLERO A, CHIO M D I, KOVACS Z S, RIZZI P, SATTA M, ZIGGIOTTI A. Thermal stability and hardness of Mg-Cu-Au-Y amorphous alloys [J]. Journal of Alloys and Compounds, 2007, 434/435: 183-186.
[6] PARK E S, KYEONG J S, KIM D H. Enhanced glass forming ability and plasticity in Mg-based bulk metallic glasses [J]. Mater Sci Eng A, 2007, 449/451: 225-229.
[7] GENG Jia-yuan, SUN Yu-feng, WANG Li-guo, ZHU Shi-jie, LIU Li-zhao, GUAN Shao-kang. Effect of Al addition on formation and mechanical properties of Mg-Cu-Gd bulk metallic glass [J]. Trans Nonferrous Met Soc China, 2007, 17(5): 907-912.
[8] GUN B, LAWS K J, FERRY M. Superplastic ?ow of a Mg-based bulk metallic glass in the supercooled liquid region [J]. Journal of Non-crystalline Solids, 2006, 352: 3896-3902.
[9] GEBERT A, HAEHNEL V, PARK E S, LEE H, KIM N J. Corrosion behaviour of Mg65Cu7.5Ni7.5Ag5Zn5Gd5Y5 bulk metallic glass in aqueous environments [J]. Electrochimica Acta, 2008, 53: 3403-3411.
[10] PAN D G, LIU W Y, ZHANG H F, WANG A M. Mg-Cu-Ag-Gd-Ni bulk metallic glass with high mechanical strength [J]. Journal of Alloys and Compounds, 2007, 438: 142-144.
[11] XU Y K, XU J. Ceramics particulate reinforced Mg65Cu20Zn5Y10 bulk metallic glass composites [J]. Scripta Mater, 2003, 49: 843-848.
[12] XU Ying-kun, MA Han, XU Jian, MA En. Mg-based bulk metallic glass composites with plasticity and gigapascal strength [J]. Acta Mater, 2005, 53: 1857-1866.
[13] MA H, XU J, MA E. Mg-based bulk metallic glass composites with plasticity and high strength [J]. Appl Phys Lett, 2003, 83(14): 2372-2375.
[14] HUI X, DONG W, CHEN G L, YAO K F. Formation, microstructure and properties of long-period order structure reinforced Mg-based bulk metallic glass composites [J]. Acta Materialia, 2007, 55: 907-920.
[15] ZHAO Yuan-yun, MABAND E, XU Jian. Reliability of compressive fracture strength of Mg-Zn-Ca bulk metallic glasses: Flaw sensitivity and Weibull statistics [J]. Scripta Mater, 2008, 58: 496-499.
[16] ZHANG Z F, SHEN B L, INOUE A, ECKERTJ. Shear fracture and fragmentation mechanisms of bulk metallic glasses [J]. Philosophical Magazine Letters, 2006, 86(10): 643-650.
[17] LI Z G, HUI X, ZHANG C M, CHEN G L. Formation of Mg-Cu-Zn-Y bulk metallic glasses with compressive strength over gigapascal[J]. Journal of Alloys and Compounds, 2008, 454(1/2): 168-173.
[18] TAKAGI M, KAWAMURA Y, SAKA H, IMURA T. Effect of preparation technique and atmosphere on the mechanical properties of bulk amorphous alloy compacts [J]. Mater Sci Eng A, 1991, 133: 301-306.
[19] QIN F X, BAE G T, DAN Z H, LEE H, KIM N J. Corrosion behavior of the Mg65Cu25Gd10 bulk amorphous alloys [J]. Mater Sci Eng A, 2007, 449/451: 636-639.
[20] MA H, SHI L L, XU J, LI Y, MA E. Discovering inch-diameter metallic glasses in three-dimensional composition space [J]. Appl Phy Lett, 2005, 87(18): 181915-1-3.
(Edited by YANG Hua)
Foundation item: Project(2007CB613704) supported by the National Basic Research Program of China; Project(50725413) supported by the National Natural Science Foundation of China; Project(CSTS2006BB4199) supported by the Project of CQ CSTC and the State Key Laboratory of Metastable Materials Science and Technology, Yanshan University, China
Corresponding author: WANG Jing-feng; Tel: +86-23-65112153; E-mail: jfwang@cqu.edu.cn