
Preparation and electrochemical performance of
tantalum-doped lithium titanate as anode material for lithium-ion battery
HU Guo-rong, ZHANG Xin-long, PENG Zhong-dong
School of Metallurgical Science and Engineering, Central South University, Changsha 410083, China
Received 28 October 2010; accepted 21 March 2011
Abstract: The electrochemical performance of Ta-doped Li4Ti5O12 in the form of Li4Ti4.95Ta0.05O12 was characterized. X-ray diffraction (XRD) and scanning electron microscopy (SEM) were employed to characterize the structure and morphology of Li4Ti4.95Ta0.05O12. Ta-doping does not change the phase composition and particle morphology, while improves remarkably its cycling stability at high charge/discharge rate. Li4Ti4.95Ta0.05O12 exhibits an excellent rate capability with a reversible capacity of 116.1 mA·h/g at 10C and even 91.0 mA·h/g at 30C. The substitution of Ta for Ti site can enhance the electronic conductivity of Li4Ti5O12 via the generation of mixing Ti4+/Ti3+, which indicates that Li4Ti4.95Ta0.05O12 is a promising candidate material for anodes in lithium-ion battery application.
Key words: lithium-ion battery; lithium titanate; anode material; doping
1 Introduction
The ever-growing demand for portable batteries with high energy density is exerting pressure for the development of advanced lithium-ion battery. For large-scale applications such as electric and hybrid vehicle systems, the vital issue is the availability of advanced materials. In recent years, there has been considerable effort devoted to developing high energy density, safe and reliable new materials to use as the anodes in lithium-ion battery [1]. The spinel Li4Ti5O12 has been found to be an attractive anode material for lithium-ion battery [2-4]. In the lithium titanate spinel-type structure of Li4Ti5O12, the formal valence of titanium is +4, which is the highest achievable oxidation state possible for titanium. This Li4Ti5O12 material has been found to intercalate lithium ions without strain or shrinkage to the lattice. It has a flat Li insertion potential at about 1.55 V (versus Li+/Li), above the reduction potential of common electrolyte solvents, mitigating the formation of solid-electrolyte interface (SEI) and avoiding formation of lithium dendrites to make the battery safer. However, several disadvantages exist compared with graphite, including the poor electric conductivity that limits its full capacity at high charge-discharge rates.
Effort has been expended to improve the conductivity. Several methods were proposed: 1) doping Li4Ti5O12 with other metal cations or non-metal ions in Li, Ti or O sites [5-11]; 2) incorporating a second phase with high electronic conductivity such as carbon and metal powder [12-14]; 3) making a nitridation to form oxynitride species on its surface [15]. However, to our best knowledge, there are less investigations on the electrochemical characteristics of Ta-doped Li4Ti5O12 as an anode material. In this work, we proposed to partially substitute Ti4+ with Ta5+, which will cause a transition of a certain amount of Ti4+ to Ti3+ as charge compensation. The transition from Ti4+ to Ti3+ in Li4Ti5O12 will lead to an increase in the electronic conductivity and thus improve the rate performance.
2 Experimental
2.1 Preparation of materials
Li4Ti5O12 samples were prepared using a solid-state method from TiO2 (anatase structure) and LiOH·H2O. The Ta-doped Li4Ti5O12 was prepared also using a solid-state method with TiO2 (anatase structure), LiOH·H2O and Ta2O5. In both cases, mass fraction excess LiOH·H2O was used to compensate for lithium volatilization during the high temperature heating. A doping level of 1% (mole fraction) Ta was selected, which yields the following composition, Li4Ti4.95Ta0.05O12, assuming that Ta sits on a Ti site. This is probably a good assumption in that the ionic radius of Ta5+ is 0.064 nm, similar to the ionic radius of Ti4+ (0.061 nm) and much smaller than the ionic radius of Li+ (0.076 nm). Powders of the precursor materials were mixed in a mortar and pestle with enough methanol to form slurry. The undoped mixed reactant mixture was heated at 800 °C for 5 h in air (oxidizing) and the Ta-doped mixed reactant mixture was heated at 800 °C for 5 h in 3% (volume fraction) H2/Ar (reducing) to obtain the final powders.
2.2 Characterization
The crystal structure of the powders was characterized by X-ray diffraction (XRD, Rigaka Dmax-RB) using Cu-Kα radiation (10°≤2θ≤90°). Cell parameters were refined with the program FULLPROF [16]. Scanning electron microscope (SEM, KYKY2800) was used to study the morphology of the materials.
2.3 Electrochemical tests
The electrochemical cycling performances of the Li4Ti5O12 powders were evaluated at room temperature (20 °C) with laboratory-scale Li/Li4Ti5O12 button cells including a lithium metal foil as counter electrode, a composite of 80% (mass fraction) Li4Ti5O12, 10% (mass fraction) acetylene black (AB), and 10% (mass fraction) polytetrofluornethelene (PTFE) binder as a cathode. A micro-porous polypropylene film (Celgard 2400) was used as a separator and 1 mol/L LiPF6 solution with the 1:1 in volumetric ratio of ethylene carbonate to dimethyl carbonate (EC-DMC) was used as the electrolyte. All cells were assembled inside a glove box filled with ultra-pure argon. Charge-discharge characteristics of the cells were recorded in the potential range of 1.0-3.0 V using a LAND batteries test system (CT2001A, Jinnuo Electronics Co., Ltd., Wuhan, China) and specific capacities were calculated based on the mass of Li4Ti5O12. Electrochemical impedance spectrum (EIS) was also measured using a potentiostat/galvanostat EG&G 273A coupled to a frequency response analyzer (FRA) EG&G 1025. The impedance data were obtained between 1 MHz and 10 mHz at 5 mV as the applied sinusoidal perturbation. Electrical resistance measurement of the sintered and polished pellets of Li4Ti5O12 was also performed at electrochemical workstation above.
3 Results and discussion
3.1 Phase characteristics
The color of the synthesized Li4Ti5O12 powder is white. The XRD patterns of the Li4Ti5O12 and Li4Ti4.95Ta0.05O12 are shown in Fig. 1(a). All the sharp diffraction peaks can be attributed to the cubic spinel structure of Li4Ti5O12 without obvious impurity phase, which indicates that Ta5+ has successfully entered the lattice of the spinel and do not change its structural characteristics. The XRD pattern reveals that the (111) peak of the Li4Ti4.95Ta0.05O12 shifted to smaller angels. For a clear observation, the peak position variation of (111) plane is magnified and shown in Fig. 1(b). The XRD refinement according to the Rietveld method indicates that the lattice parameter of Li4Ti4.95Ta0.05O12 is 8.368 ? and that of the Li4Ti5O12 is 8.362 ? [17], which are consistent with results reported by WOLFENSTINE and ALLEN [10]. The doping of Ta will cause the lattice constant to become large. This may be caused by the reason that the substitution of Ta5+ for Ti4+ site will cause the transition of a certain amount of Ti4+ to Ti3+ as charge compensation [10], which will cause the increase of the lattice constant of the Li4Ti5O12 because Ti3+ (0.067 nm) is larger than Ti4+ (0.061 nm). For heat-treatment under reducing conditions, according to charge neutrality condition (Kroger-Vink notation [16]) is given in Eq. (1) and hence, an increase in electronic conductivity occurs [10].
[e′]=[TaTi·] (1)
where an electron, e′, corresponds to Ti3+ on a Ti4+ site.
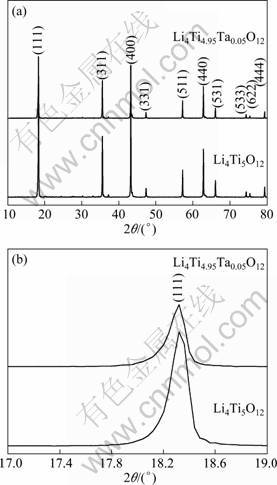
Fig. 1 XRD patterns of Li4Ti5O12 and the Li4Ti4.95Ta0.05O12 (a) and magnified (111) peaks of Li4Ti5O12 and Li4Ti4.95Ta0.05O12 (b)
3.2 Morphology
Figure 2 shows the SEM images of the undoped Li4Ti5O12 powders and the Ta-doped powders. The grains of pure Li4Ti5O12 are small with the size generally distributed in the range of 200-300 nm. Small particles will enlarge the contact areas between grains and electrolyte, and thus improve the specific capacity of the electrode. In Fig. 2(b), the grain size of the Ta-doped powders is larger than the pure one, but is also nano-sized.
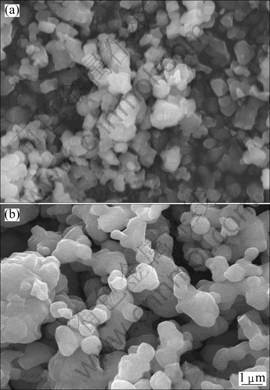
Fig. 2 SEM images of Li4Ti5O12 (a) and Li4Ti4.95Ta0.05O12 (b)
The acquisition of these homogeneously nano-sized Li4Ti5O12 powders could be attributed to the precursor material, nano-sized anatase structure TiO2, which could be mixed more sufficiently with the other materials compared with the usually used micro-sized rutile structure TiO2. The sintering time in this work is much shorter than the ordinarily reported 10-15 h. Short sintering time was also critical to prevent the grains from excessive growth.
3.3 Electrochemical performance
The electrochemical properties of the powders are determined by charge-discharge test at constant current density. Figure 3 shows the first charge-discharge curves of the undoped and Ta-doped Li4Ti5O12 powders at 0.1C rate in potential window between 3.0 and 1.0 V. The cycling behavior is typical of LTO with a flat plateau at an average potential of 1.55 V which is attributed to a two-phase phenomenon pertaining to Li4Ti5O12 and Li7Ti5O12 phases. The voltage platform is the result of an adjustment of the above intercalation and deintercalation processes. The sharp and linear increases of the voltage at the end of the charge and the start of the discharge curves are results of electrode polarization. The cation distribution in Li4Ti5O12 and Li7Ti5O12 phases during electrochemical charge-discharge processes could be written as follows [2]:
[Li]8a[Li1/3Ti5/3]16d[O4]32e + Li+ + e=
[Li2]16c[Li1/3Ti5/3]16d[O4]32e (2)
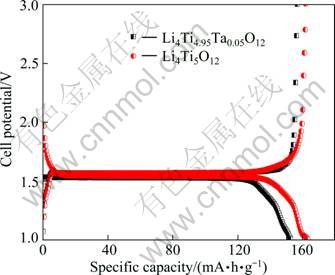
Fig. 3 Initial discharge-charge curves of Li4Ti5O12 and Li4Ti4.95Ta0.05O12 at 0.1C rate
During the first discharge, undoped Li4Ti5O12 takes about 2.78 Li atoms, corresponding to a capacity of 161.9 mA·h/g that matchs well with the expected theoretical capacity for Li4Ti5O12. However, the Ta-doped Li4Ti5O12 takes about 2.69 Li atoms, corresponding to a capacity of 156.7 mA·h/g, less than the undoped one. A small electrode polarization of about 0.02 V is observed between charge-discharge curves, indicating the existence of good interparticle electrical contacts and ion transport. In Li4Ti5O12 structure, 75% Li+ ions locate at tetrahedral 8a sites, 25% Li+ and Ti4+ are randomly distributed at octahedral 16d sites, and O2 ions occupy the 32e sites, the 8b, 48f and 16c sites are empty [2]. During lithiation process, 3 Li-ions can be accommodated by Li4Ti5O12, which will make spinel Li4Ti5O12 transform to rock-salt Li7Ti5O12. In this process, all Li-ions, including those in 8a site and the newly inserted ones, will move and occupy the 16c site, and
then all octahedral sites are filled. Ta-doping may impede the transportation of Li-ions from 8a to 16c sites, influencing the insertion of outside Li-ions into Li4Ti5O12 structure. As a consequence, the capacity of Ta-doped Li4Ti5O12 is decreased.
High rate performance is one of the most important electrochemical characteristics of lithium ion batteries for HEV application. The charge-discharge capacities of pure Li4Ti5O12 and Ta-doped Li4Ti5O12 electrodes at different current rates from 0.1C to 30C are shown in Fig. 4. It can be seen that, the rate performance of Li4Ti5O12 can be improved by doping Ta. The charge and discharge capacities decrease with increasing the current rate. Li4Ti4.95Ta0.05O12 exhibits an excellent rate capability compared with Li4Ti5O12, although Li4Ti5O12 shows much more initial capacity than Li4Ti4.95Ta0.05O12. At 0.1C, Li4Ti4.95Ta0.05O12 presents a discharge capacity of 152.8 mA·h/g, while the Li4Ti5O12 exhibits a discharge capacity of 161.9 mA·h/g. At 30C, the discharge capacity of Li4Ti4.95Ta0.05O12 still remains 91.0 mA·h/g, while the capacity of the undoped Li4Ti5O12 is only 44.5 mA·h/g. We proposed to partially substitute Ti4+ with Ta5+, which will cause a transition of a certain amount of Ti4+ to Ti3+ as charge compensation. The transition from Ti4+ to Ti3+ in Li4Ti5O12 will lead to an increase in the electronic conductivity and thus improve the rate performance.
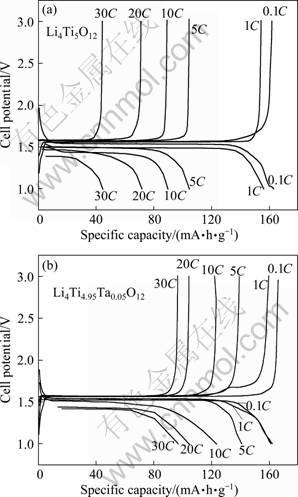
Fig. 4 Initial discharge-charge curves of Li4Ti5O12 (a) and Li4Ti4.95Ta0.05O12 (b) at different current rates (0.1C, 1C, 5C, 10C, 20C, 30C)
The cycling performance of the Ta-doped and undoped Li4Ti5O12 samples at the rate of 1C is exhibited in Fig. 5. In Fig. 5, more than 11.1% of the capacity loss occurs to the undoped Li4Ti5O12 at 1C after 100 cycles, while the Ta-doped Li4Ti5O12 loses only 6.3% after 100 cycles. The 100th discharge capacity of the Ta-doped Li4Ti5O12 electrode is 146.8 mA·h/g, but the corresponding value of the Li4Ti5O12 electrode is decreased to 144.7 mA·h/g. This indicates that the introduction of Ta ions can increase the cycle stability. This suggests that the electrode polarization is probably decreased by Ta-doping.
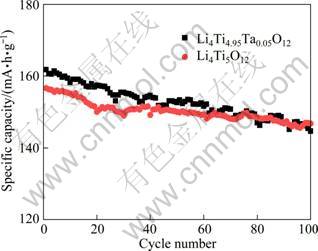
Fig. 5 Cycling performance of Li4Ti5O12 and Li4Ti4.95Ta0.05O12 samples (current rate of 1C)
Electrochemical impedance spectroscopy (EIS) may be considered one of the most sensitive tools for studying the changes in the electrode behavior due to surface modification. EIS results of the coin cells with the Li4Ti5O12 and Ta-doped Li4Ti5O12 cathodes are shown in Fig. 6. The measurement was carried out after the cells have been discharged to the depth of 50% followed by three cycles. The impedance spectra are composed of one semicircle at higher frequencies followed by linear part at lower frequency end. The semicircle in the high region represents the migration of the lithium ions at the electrode/electrolyte interface. The low frequency region of the straight line is attributed to the diffusion of the lithium ions into the bulk of the electrode material, the so-called Warburg diffusion [18].
The relationship between the imaginary impedance and the low frequencies is governed by Eq. (3). The diffusion coefficients of the lithium ions in the bulk electrode materials were calculated by Eq. (4). The Warburg impedance coefficient can be obtained from the straight lines [17-18]. The relation is governed by Eq. (5). These calculated results are recorded in Tabe1. The real impedance of the Li4Ti5O12 and the Li4Ti4.95Ta0.05O12 samples in the low frequencies is illustrated in Fig. 7.
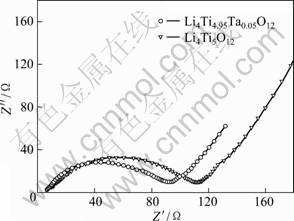
Fig. 6 EIS results of Li4Ti5O12 and Li4Ti4.95Ta0.05O12 samples
Z′′=-σwω-1/2 (3)
D=0.5
2 (4)
Zre=Rs+Rct+σwω-1/2 (5)
where Z′′ is the imaginary impedance; ω is the angular frequency in the low frequency region; Rct is the charge-transfer resistance; Rs is the electrolyte resistance; D is the diffusion coefficient; R is the gas constant; T is the thermodynamic temperature; F is the Faraday’s constant; A is the area of the electrode surface; c is the molar concentration of Li+; δw is the Warburg impedance coefficient [18-19].
Table 1 Impedance parameters of Li4Ti5O12 and Li4Ti4.95Ta0.05O12 samples
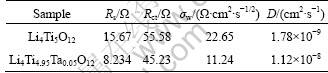
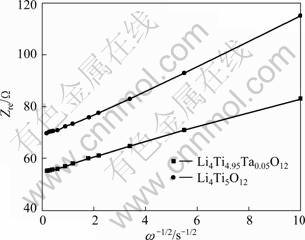
Fig. 7 Real impedance of Li4Ti5O12 and Li4Ti4.95Ta0.05O12 samples in low frequencies
The obtained diffusion coefficients show that Ta-doped Li4Ti5O12 sample has higher mobility for Li+ diffusion than the undoped Li4Ti5O12 sample. This is attributed to the concentration of electrons caused by the transition of a certain amount of Ti4+ to Ti3+ as charge compensation. The transition from Ti4+ to Ti3+ in Li4Ti5O12 will lead to an increase in the electronic conductivity and thus improve the rate performance.
4 Conclusions
1) Li4Ti4.95Ta0.05O12 powders have been successfully synthesized by a simple solid state reaction. XRD patterns show that the Li4Ti4.95Ta0.05O12 has a good crystallinity and a high phase purity.
2) The Li4Ti4.95Ta0.05O12 electrode presents better cycling performance than the Li4Ti5O12 electrode prepared by the similar process. Ta-doping does not change the phase composition and particle morphology, while improves remarkably its cycling stability at high charge-discharge rate.
3) The substitution of Ta for Ti site can enhance the electronic conductivity of Li4Ti5O12 via the generation of mixing Ti4+/Ti3+. At 30C, the discharge capacity of the Li4Ti4.95Ta0.05O12 still remains at 91.0 mA·h/g, while the capacity of the undoped Li4Ti5O12 is only 44.5 mA·h/g. More than 11.1% of the capacity loss occurs to the undoped Li4Ti5O12 at 1C after 100 cycles, while the Ta-doped Li4Ti5O12 loses only 6.3% after 100 cycles. The 100th discharge capacity of the Ta-doped Li4Ti5O12 electrode is 146.8 mA·h/g, but the corresponding value of the Li4Ti5O12 electrode is decreased to 144.7 mA·h/g.
References
[1] TARASCON J M, ARMAND M. Issues and challenges facing rechargeable lithium batteries [J].Nature, 2001, 414: 359-367.
[2] OHZUKU T, UEDA A, YAMAMOTO N. Zero-strain insertion material of Li[Li1/3Ti5/3Ti5/3]O4 for rechargeable lithium cells [J]. Journal of the Electrochemical Society, 1995, 142: 1431-1435.
[3] JIANG C, ICHIHARA M,HONMA I, ZHOU H.
Effect of particle dispersion on high rate performance of nano-sized Li4Ti5O12 anode [J]. Electrochimica Acta, 2007, 52: 6470-6475.
[4] ARIYOSHI K, YAMATO R, OHZUKU T.Zero-strain insertion mechanism of Li[Li1/3Ti5/3]O4 for advanced lithium-ion (shuttlecock) batteries [J]. Electrochim Acta, 2005, 51: 1125-1129.
[5] CHEN C H, VAUGHEY J T, JANSEN A N, DEES D W, KAHAIAN A J, GOACHER T, THACKERAY M M. Studies of Mg-substituted Li4-xMgxTi5O12 spinel electrodes for lithium batteries [J]. J Electrochem Soc A, 2001, 148: 102-104.
[6] ROBERTSON A D, TREVINO L, TUKAMOTO H, IRVINE J T S. New inorganic spinel oxides for use as negative electrode materials in future lithium-ion battery [J]. J Power Sources, 1999, 81-82: 352-357.
[7] MARTLN P, LOPEZ M L, PICO C, VEIGA M L. Li(4-x)/3Ti(5-2x)/3CrxO4 (0 ≤ x ≤ 0.9) spinels: New negatives for lithium batteries [J]. Solid State Sci, 2007, 9: 521-526.
[8] ROBERTSON A D, TUKAMOTO H, IRVINE J T S. Li1+xFe1-3xTi1+2xO4 (0.0≤x≤0.33) based spinels: Possible negative electrode materials for future Li-ion batteries [J]. J Electrochem Soc, 1999, 146: 3958-3962.
[9] ZHONG Z. Synthesis of Mo4+ substituted spinel Li4Ti5-xMoxO12 [J]. Electrochem Solid-State Lett A, 2007, 10: 267-269.
[10] WOLFENSTINE J, ALLEN J L. Electrical conductivity and charge compensation in Ta doped Li4Ti5O12 [J]. J Power Sources, 2008, 180: 582-585.
[11] ALLEN J L, JOW T R, WOLFENSTINE J. Low temperature performance of nanophase Li4Ti5O12 [J]. J Power Sources, 2006, 159: 1340-1345.
[12] DUBIW V M, DIAMANG Y S, ZHAO B, VASUDEV P K, TING C H. Selective and blanket electroless copper deposition for ultralarge scale integration [J]. J Electrochem Soc, 1997, 144: 898-908.
[13] KAVAN L, DUNSCH L, KATAURA H. Electrochemical tuning of electronic structure of carbon nanotubes and fullerene peapods [J]. Carbon, 2004, 42: 1011-1019.
[14] HUANG S, WOODSON M, SMALLEY R, LIU J. Growth mechanism of oriented long single walled carbon nanotubes using "fast-heating" chemical vapor deposition process [J]. Nano Lett, 2004, 4: 1025-1028.
[15] PARK K S, BENAYAR A, KANG D J, DOO S G. Nitridation-driven conductive Li4Ti5O12for lithium ion batteries [J]. J Am Chem Soc, 2008, 130: 14930-14931.
[16] BARSUM M V. Fundamentals of ceramics [M]. New York: The McGraw-Hill Companies, Inc, 1997: 137-161.
[17] RODRIGUEZ C J. Recent advances in magnetic structure determination by neutron powder diffraction [J].Physica B, 1993, 192: 55-69.
[18] SHENOUDA A Y, LIU H K. Electrochemical behaviour of tin borophosphate negative electrodes for energy storage systems [J]. J Power Sources, 2008, 185: 1386-1391.
[19] BARD A J, FAULKNER L R. Electrochemical methods [M]. New York: John Wiley & Sons, 2001: 376-388.
锂离子电池极材料钽掺杂钛酸锂的
制备及电化学性能
胡国荣, 张新龙, 彭忠东
中南大学 冶金科学与工程学院,长沙 410083
摘 要:通过固相合成制备了钽掺杂材料 Li4Ti4.95Ta0.05O12。通过XRD和SEM来表征 Li4Ti4.95Ta0.05O12的结构和形貌。 钽掺杂并没有改变本体材料的结构和形貌,而且显著提高了材料的循环性能和倍率性能。Li4Ti4.95Ta0.05O12 在10C和30C倍率时的放电容量分别是116.1 mA·h/g 和 91.0 mA·h/g。Ta掺杂取代了Li4Ti5O12中的Ti的位置,产生了Ti4+/Ti3+混合价态,从而提高了钛酸锂的电导率。故具有优异的高倍率性能,是一种优异的锂离子电池负极材料。.
关键词:锂离子电池;钛酸锂;负极材料;掺杂
(Edited by YANG Hua)
Corresponding author: HU Guo-rong; Tel: +86-731-88830532; E-mail: hgrhsj@263.net
DOI: 10.1016/S1003-6326(11)61003-0