J. Cent. South Univ. (2017) 24: 1245-1251
DOI: 10.1007/s11771-017-3528-3

Effect of process parameters on microstructural and mechanical properties of Ti-SS 304L explosive cladding
Saravanan Somasundaram1, Raghukandan Krishnamurthy2, Hokamoto Kazuyuki3
1. Department of Mechanical Engineering, Annamalai University, Annamalai Nagar, Tamilnadu 608002, India;
2. Department of Manufacturing Engineering, Annamalai University, Annamalai Nagar, Tamilnadu 608002, India;
3. Institute of Pulsed Power Science, Kumamoto University, Kurokami, Kumamoto, Japan
Central South University Press and Springer-Verlag Berlin Heidelberg 2017
Abstract: The present work pertains to the study of microstructure and mechanical properties of explosively cladded commercially pure titanium (cpTi) with austenitic stainless steel (SS 304L) subjected to varied process parameters, viz., loading ratios (mass of explosive or mass of flyer plate) and preset angles. The microstructural study reveals the transformation from straight to wavy interface while increasing loading ratios. Vickers hardness increases with loading ratios, and the maximum hardness is witnessed in the closer proximity of collision interface. Ram tensile and shear strength of explosive claddings are higher than that of parent plates. While the base plate fails in impact test, the flyer plate is deformed, indicating good impact strength. Increased mass of claddings, due to oxide formation, is witnessed in corrosion tests, which confirms the superiority of explosive claddings in corrosive environment. Triaxial weldability window, an analytical estimation for Ti-SS 304L explosive claddings, is developed and correlated.
Key words: explosive cladding; titanium; stainless steel; microstructure; strength; corrosion
1 Introduction
Titanium and its alloys are employed in the constructions of reactors, autoclaves, infrastructures, periphery applications, crystallizers and pressure vessels owing to their remarkable corrosion resistance and specific strength [1]. When higher thickness is essential in economical means, claddings of other metals with titanium are inevitable and attempted by earlier researchers [2-4]. Titanium clad stainless steel is preferred over other combinations, when corrosion resistance, strength and cost are necessitated. Titanium clad steel plates combine the corrosion resistance of titanium with the high strength and low cost of stainless steel substrate. Nonetheless, conventional welding of titanium and steel is challenging due to the high reactivity of titanium with oxygen and nitrogen leading to the formation of brittle intermetallic compounds at high temperature [5].
Explosive cladding, a solid state welding technique, offers a reliable solution to clad titanium on stainless steel plates, whose process parameters, viz., nature of explosive, loading ratios (mass of explosive or mass of flyer plate), preset angles and stand-off distance dictate the claddings’ microstructure, and consequently the mechanical strength of claddings [6]. The characteristic wavy interface is as strong as or stronger than that of the participant metals [7]. The objective of this study is to fabricate explosively cladded cpTi-SS 304L plates based on pressure vessel for purified terephthalic acid and autoclaves used in pressure acid leaching of metal ores operating at high temperatures and to determine the mechanical properties of the explosive claddings subjected to varied loading ratios and preset angles. Microstructural, corrosion and mechanical properties, viz., Vickers microhardness, ram tensile, ASTM side shear and impact test on cpTi-SS 304L explosive claddings are determined as relevant standards and the results are presented. In addition, a triaxial weldability window, an analytical estimation for determining the nature of interface for Ti-SS 304L explosive cladding, is developed.
2 Experimental procedure
Inclined explosive cladding configuration reported elsewhere [8] was attempted with commercially pure titanium (60 mm×80 mm×3.5 mm) and stainless steel 304L (60 mm×80 mm×9 mm) as flyer and base plates, respectively. The chemical composition and mechanical properties of participant metals are given in Tables 1 and 2, respectively. Nitroglycerine explosive with detonation velocity of 2800 m/s and density of 2.3 g/cm3 was the energy generator. The loading ratio, R, was varied from 0.75 to 1.75, whereas the preset angle, A, was ranged from 3° to 8°. A stand-off distance of 5 mm between flyer and base plate was maintained for all experiments.
Table 1 Composition of participant metals
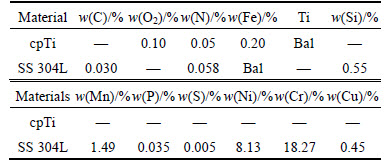
Table 2 Mechanical properties of participant metals
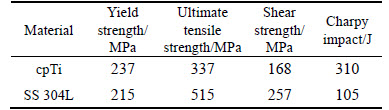
The specimens for metallographic observations were sectioned parallel to the detonation direction following standardized metallurgical procedures, viz., grinding, polishing and etching, and the post cladding metallographic analysis was conducted in a VERSAMAT–3 with Clemex image analyzing system and scanning electron microscope (JEOL, JSM–6610LV) equipped with energy dispersive spectroscopy (EDS). In addition, the chemical phase of clad was determined through X-ray diffraction analysis, using Cu Kα radiation with a 0.02°/min scan speed in an automated Rigaku RINT 2500V diffractometer, for phase identification.
Vickers microhardness measurement across the explosive claddings was carried out followed ASTM E384 standard on a ZWICK microhardness tester with a 4.9 N load and the results were presented. Two sets of ram tensile test specimens (32 mm×24 mm), for each experimental condition, were prepared in the direction of detonation (Figs. 1(a) and 1(b)), and the tests were performed in a servo controlled universal testing machine (UNITEK-94100) in compression mode with a 0.5 mm/min cross-head speed and the average values were reported. Impact tests specimens followed ASTM E23 standard (Fig. 2(a)) having a (45± 2)° V-notch of 0.2 mm fillet radius and 2 mm depth (Fig. 2(b)) were machined along the middle section of the Ti-SS 304L explosive cladding and the tests were conducted in a charpy impact testing machine (ENKAY-SM 30) at room temperature (25 °C). Three sets of impact samples were fractured for each condition and average values were presented. The impact fracture surface was analyzed with a scanning electron microscope (JEOL, JSM–6610LV) to determine the nature of fracture.
The side shear test samples were fabricated followed ASTM B898-99 standard (Fig. 3(a)) with a compressive force applied on the Ti-SS 304L explosive cladding as shown in Fig. 3(b). To study the corrosion behavior, samples (15 mm×15 mm) were placed in a glass of 3.5% NaCl aqueous solution for 720 h and the variation in mass was observed in an electronic scale.
3 Results and discussion
3.1 Microstructural characterization
The microstructural observation of the titanium- stainless steel explosive claddings shows wavy morphologies as reported by earlier researchers [9-11]. When the loading ratio, R, and preset angle, A, increase, the waves at interfaces are more pronounced. The undulating interfaces are characteristics of explosive cladding process, and are influenced by the system parameters, viz., nature of explosive, stand-off distance and preset angle. The Ti-SS 304L explosive claddings are sound, devoid of defects, viz., cracks, trapping of jet and molten layered zones are at lower energetic conditions, though formation of trapped jet and continuous molten layer are witnessed at a higher loading ratio (R=1.75) because of a higher kinetic energy dissipation and pressure developed.
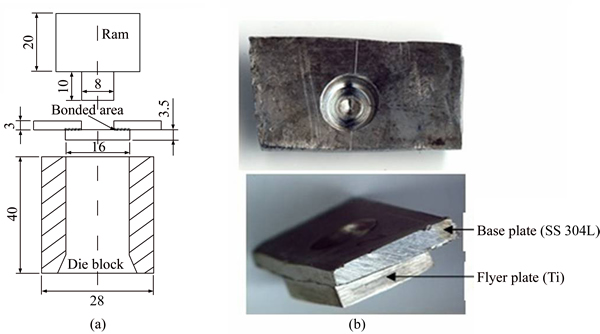
Fig. 1 Ram tensile setup (a) and specimen (b) (unit: mm)
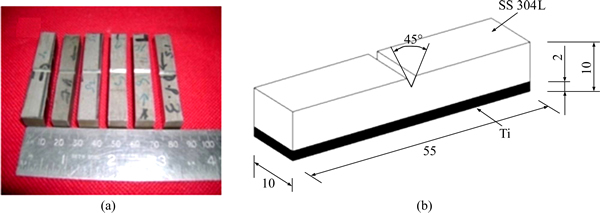
Fig. 2 Impact specimens (a) and dimensions of impact specimens (b) (unit: mm)
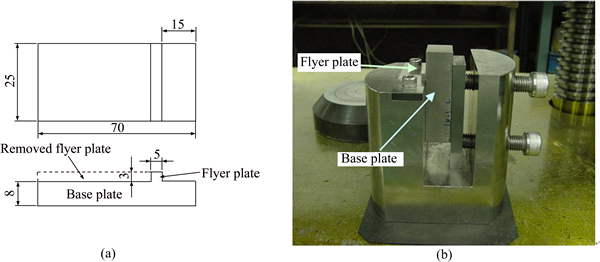
Fig. 3 Dimensions of shear test sample (a) and experimental setup (b) (unit: mm)
The microstructures of Ti-SS 304L explosive claddings for the loading ratio of 0.75 are shown in Figs. 4(a) and (b). At the lower energetic conditions, the flyer plate and collision velocities are diminutive, and the energy spent for plastic deformation is just adequate enough to craft smaller waves to resemble like a straight interface with minimal amplitude and wavelength. When the explosive loading ratio, R, is increased to 1.25, the metal flow around the collision point becomes unstable and oscillates due to the enhanced energy available and deformation work done, and creates a wavy interface with the higher interfacial amplitude and wavelength(Figs. 4(c) and (d)) as reported by TAMILCHELVAN et al [12]. When the loading ratio is further increased to 1.75, the collision velocity, plate velocity and pressure developed increase further following the higher kinetic energy spent at the Ti/SS 304L interface to result in the highest interfacial amplitude and wavelength (amplitude of 75 μm, wavelength of 375 μm).
In addition, the formation of ‘trapped jet’ and continuous molten layer is witnessed at the interface for preset angles of 3°(Fig. 4(e)) and 8°(Fig. 4(f)), respectively. Formation of solidified melt as a result of rapid heat transfer is due to excess kinetic energy dissipation when the loading ratio, R, is increased as reported by MANIKANDAN et al [13]. The unescaped jet manifests into a trapped jet, either partially or completely, in the vortices formed, which is consistent with that BORCHERS et al [14] reported.
ABE [15] stated that due to deflection of surface jet, the palstic strain is experienced largely on the lower density metal and contributes to the formation of assymetric waves as observed in Fig. 5(a). SEM images along with EDS images mapping across the vortex (Figs. 5(b) and (c)) indicate a uniform sharing of iron and titanium elements due to vigorous mixing of the “surface jet” and the higher cooling rate experienced by the molten metal. The elemental mapping images indicates the formation of iron rich layer at the vortex since the distribution of iron is prominent in the vortex (57%Fe,18%Ti, 17.5%Cr, 6.5%Ni, 1%Si, molar fraction). These contents of iron and titanium are located in the eutectic region of phase diagram which promotes the formation of intermetallic compounds FeTi and Fe2Ti. The XRD results (Fig. 6) confirm the presence of both FeTi and Fe2Ti intermetallic compounds dispersed in the vortex.
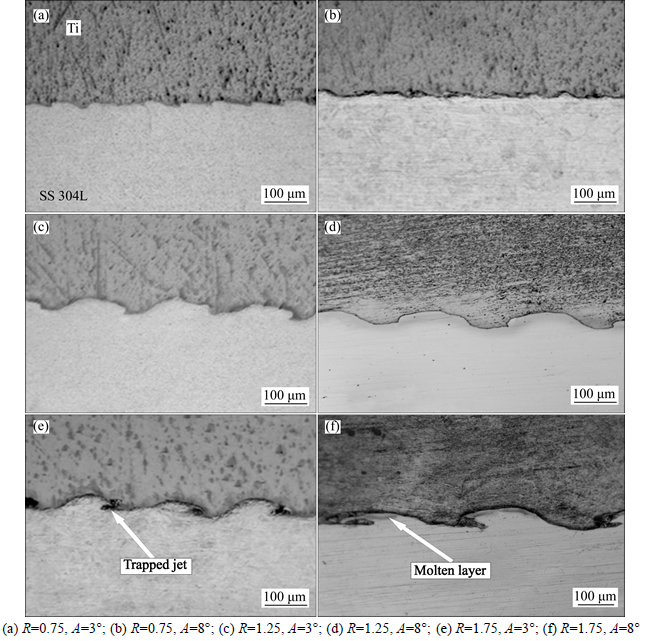
Fig. 4 Microstructures of Ti-SS 304L explosive claddings:
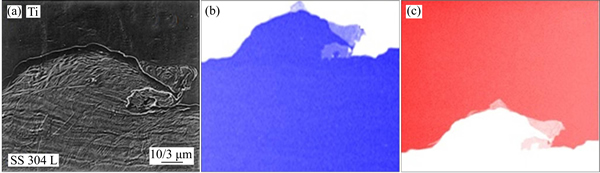
Fig. 5 SEM image of Ti/SS 304L interface (a), Fe distribution (b) and Ti distribution (c)
3.2 Mechanical strength
3.2.1 Microhardness variation
The variation in Vickers microhardness plot for the Ti-SS 304L explosive claddings is shown in Fig. 7. For each sample, three measurements are taken and the average values are reported. The average hardness of base plate and flyer plate are HV 252 and HV 182, respectively, owing to their martensitic and α structure.
The Vickers microhardness of interface increases to HV 200, HV 215 and HV 227 for loading ratios of 0.75, 1.25 and 1.75, respectively. Microhardness of the interface increases with loading ratio, R, following the higher plate velocity, collision velocity and pressure developed due to impact. The increase in hardness of zones close to the interface is proportional to loading ratio as reported by HONARPISHEH et al [16]. It is also observed that there is no significant variation away from the interface. The peak hardness of HV 318 is achieved on SS 304L side of the claddings (around 100 μm) near the interface due to tempered bainite or martensite nature of microstructure. Maximum hardness is obtained near the interface due to maximum deformation work spent adjacent to the interface following high level of plastic deformation and energy spent at the interface.
and ACARER [17] joined duplex steel with steel at varied loading ratios and found that zones near the collision interface exhibited higher hardness at a higher explosive load, which was consistent with the results of this study.
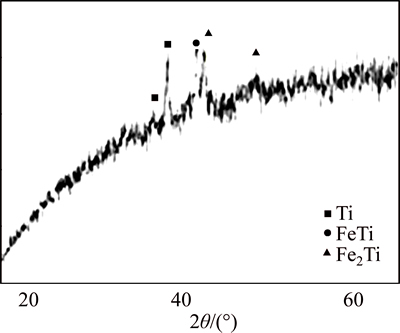
Fig. 6 XRD patterns of Ti-SS 304L explosive cladding interface
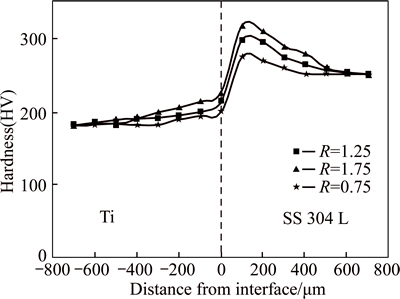
Fig. 7 Hardness profile of Ti-SS 304L explosive claddings
3.2.2 Ram tensile test
During testing, the weaker metal (titanium) was detached from SS 304L for all experimental conditions, which did not occur in the Ti-SS 304L explosively cladded interface, thereby indicating that the bond strength is higher. The maximum tensile strength of Ti-SS 304L explosive claddings is 286 MPa (Table 3), which is close to tensile strength of SS 304L. The tensile strength of claddings increases with loading ratio, R and preset angle, A.
Table 3 Ram tensile and shear strength of Ti-SS 304L explosive claddings
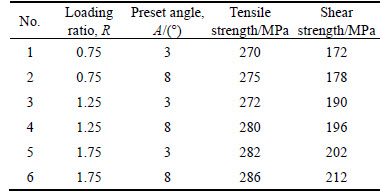
The maximum tensile strength is achieved by the cladding having smooth undulating interfaces when loading ratio, R, and preset angle, A, are higher, and the enhancement in contact area with higher amplitude and wavelength leads to a better mechanical strength. However, the least tensile strength is obtained for an interface that is straight, though it is found to be higher than that of the parent metal (Ti, 170 MPa). It can be inferred that Ti-SS 304L explosive cladding can effectively be employed in industrial applications which demands good strength. Though wavy interfaces are preferred, straight interfaces also results in acceptable strength as reported by KAHRAMAN et al [2]. WRONKA [18] reported that under perfect cladding conditions, the tensile strength of the claddings was superior to that of the participant metals.
3.2.3 ASTM side shear test
The compressive force is applied on the Ti-SS 304L explosive claddings by measuring load with respect to displacement, until the sample fails on Instron universal testing machine at 0.5 mm/min and the results is shown in Table 3. The shear strength of claddings is higher than that of titanium for all experimental conditions, but are lower than that of SS 304L, indicating that the claddings possess acceptable shear strength. The maximum shear strength is obtained at a higher loading ratio owing to the formation of undulating interfaces following a higher kinetic energy spent at the interface. The shear strength of the claddings are 63%-75% of the tensile strength of titanium and prevailing between the shear strengths of parent metals, which is consistent with the results of RAO et al [3].
3.2.4 Charpy impact test
The maximum and minimum impact-shear load released from the Ti-SS 304L explosive claddings are measured as 212 J and 195 J,respectively. The impact toughness increases with loading ratio and preset angle. During testing, specimens were fractured on the base plate, whereas the flyer plate was bent, but was not fractured (Fig. 8), indicating good strength, which is consistent with the results of KAYA and KAHRAMAN [19].
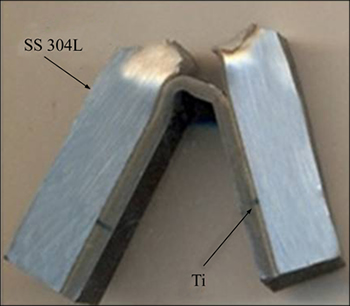

Fig. 8 Failed impact specimen
The SEM fractograph of the impact test specimen (Fig. 9) reveals the evidence for a traditional ductile fracture characteristic feature in explosive claddings. Ductile fracture surface consists of copious dimples or micro voids. Parabolic shaped dimples with its tip point out the direction of shear. The dimple fracture at the impact direction is owing to very fine grains formed at the Ti-SS 304L explosively cladded interface, which leads to a better mechanical strength.
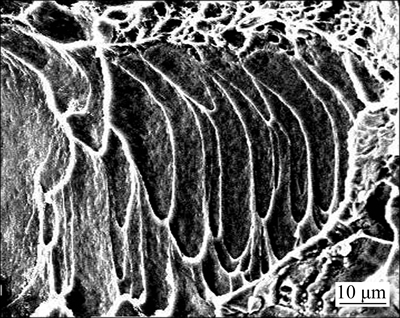
Fig. 9 SEM fractograph of impact specimen
3.3 Corrosion behaviour
The specimens were recovered from the corrosive environment and cleaned following EN ISO 7384 standards, then weighed, and the variation in the propertion between mass and surface area was determined. The mass of Ti-SS 304L explosive claddings increases for all conditions. The maximum increased proportion between mass and surface area of 0.04 g/cm2 is obtained when loading ratio and preset angle are the maximum (R=1.75, A=8°). The greater affinity of titanium to oxygen enhances the mass of thereby, contributing the enhancement in mass. The increase in mass is also attributed to the formation of layer containing iron and titanium oxide [20]. Hence it can be inferred that cpTi-SS 304L explosive claddings can be safely employed in corrosive environments such as autoclaves, pressure vesels and crystallizers.
4 Weldability window
Conventional weldability windows are constructed between any two desired parameters, which contain straight and curved boundaries to define the minimum and maximum dynamic bend angle and critical angle for jetting. MOUSAVI and SARTHANGI [9] elucidated the contributions of various researchers in determining the various boundaries viz., upper boundary, lower boundary, left and right boundaries of weldability window for the selection of optimum operational parameters to produce an acceptable cladding. Weldability window for cpTi-SS 304L explosive cladding was developed based on empirical equations reported by earlier researchers [9, 11, 21]. SARAVANAN and RAGHUKANDAN [22] concluded that the experimental conditions close to lower boundary were preferable, because a lower collision angle resulted in small waves, and lower plate velocity reduced interfacial layer and defects. While the biaxial weldability window reveals the trends and relationships between any two parameters, it is preferential to develop a triaxial weldability window, so as to incorporate three optimal parameters. To study the influence of three vital parameters viz., plate velocity, welding velocity and collision angle, an attempt is made to develop a triaxial weldability window for cpTi-SS 304L explosive claddings (Fig. 10) which provides the prevailing interrelationship between the selected three parameters instead of two in a single plot, with experimental conditions superimposed. The three dimensional lower boundary of the welding domain is generated and the other boundaries are not shown. Triaxial weldability windows simplify the complicated concepts and convey complex inter-relationships prevailing between the selected parameters suitable for designing experimental conditions.
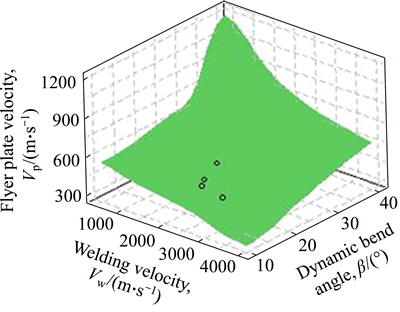
Fig. 10 Triaxial weldability window for cpTi-SS 304L explosive cladding
5 Conclusions
1) The interfacial undulations of the Ti-SS 304L explosive claddings increases with loading ratio, R and preset angle, A, following additional kinetic energy dissipation, collision pressure and plastic deformation at the interface.
2) Trapping of jet and continuous molten layer is witnessed at higher loading ratios.
3) Ram tensile strength of the claddings is higher than that of titanium, indicating the bond is stronger than that of metal.
4) Vickers microhardness of the post claddings increases with loading ratio and is higher than that of precladded metals for attempted conditions due to sudden deformation. The maximum hardness is observed close to the interface.
5) In room temperature impact toughness testing, fracture takes place in base metal, whereas flyer plate is just slightly bent, which indicates good joint strength.
6) In corrosion test, the mass of titanium increases due to oxide formation, indicating its effectiveness in corrosive environment.
7) The lower boundary of a triaxial weldability window is developed to recognize the relationship among three influencing process parameters.
References
[1] CROSSLAND B. Explosive welding of metals and its applications [M]. Oxford: Oxford University Press, 1982.
[2] KAHRAMAN N, GULENC B, FINDIK F. Corrosion and mechanical-microstructural aspects of dissimilar joints of Ti–6Al–4V and Al plates [J]. International Journal of Impact Engineering, 2007, 34(8): 1423-1432.
[3] RAO V N, REDDY M G, NAGARJUNA S. Structure and properties of explosive clad HSLA steel with titanium [J]. Transactions of Indian Institute of Metals, 2014, 67 (1): 67-77.
[4] SARAVANAN S, RAGHUKANDAN K. Diffusion kinetics in explosive cladding of dissimilar metals as described through the Miedema model [J]. Archives of Metallurgy and Materials, 2014, 59(4): 1615-1618.
[5] MANIKANDAN P, HOKAMOTO K, DERIBAS A A, RAGHUKANDAN K, TOMOSHIGE R. Explosive welding of titanium/stainless steel by controlling energetic conditions [J]. Material Transactions, 2006, 47(8): 2049-2055.
[6] ACARER M, GULENC B, FINDIK F. The influence of some factors on steel/steel bonding quality on their characteristics of explosive welding joints [J]. Journal of Materials Science, 2003, 39(21): 6457-6466.
[7] SARAVANAN S, RAGHUKANDAN K, HOKAMOTO K. Improved microstructure and mechanical properties of dissimilar explosive cladding by means of interlayer technique [J]. Archives of Civil and Mechanical Engineering, 2016, 16: 563-568.
[8] FINDIK F. Recent developments in explosive welding [J]. Materials and Design, 2011, 32(3): 1081-1093.
[9] MOUSAVI S A A A, SARTANGI P F. Experimental investigation of explosive welding of cp-titanium/AISI 304 stainless steel [J]. Materials and Design, 2009, 30(3): 459-468.
[10] SARAVANAN S, RAGHUKANDAN K. Thermal kinetics in explosive cladding of dissimilar metals [J]. Science and Technology of Welding and Joining, 2012, 17 (2): 99-103.
[11] ZAMANI E, LIAGHAT G H. Explosive welding of stainless steel-carbon steel coaxial pipes [J]. Journal of Materials Science, 2012, 47(2): 685-695.
[12] TAMILCHELVAN P, RAGHUKANDAN K, SARAVANAN S. Kinetic energy dissipation in Ti-SS explosive cladding with multi loading ratios [J]. Iranian Journal of Science and Technology- Transactions of Mechanical Engineering, 2014, 38 (M1): 91-96.
[13] MANIKANDAN P, HOKAMOTO K, FUJITA M, RAGHUKANDAN K, TOMOSHIGE R. Control of energetic conditions by employing interlayer of different thickness for explosive welding of titanium/304 stainless steel [J]. Journal of Materials Processing Technology, 2008, 195(1-3): 232-240.
[14] BORCHERS C, LENZ M, DEUTGES M, KLEIN H,
M, HAMMERSCHMIDT M, KREYE H. Microstructure and mechanical properties of medium-carbon steel bonded on low-carbon steel by explosive welding [J]. Materials and Design, 2016, 89(8): 369-376.
[15] ABE A. Numerical simulation of the plastic flow field near the bonding surface of explosive welding [J]. Journal of Materials Processing Technology, 1999, 85(S1-3): 162-165.
[16] HONARPISHEH M, ASEMABADI M, SEDIGHI M. Investigation of annealing treatment on the interfacial properties of explosive- welded Al/Cu/Al multilayer [J]. Materials and Design, 2012, 37: 122-127.
[17]
R, ACARER M. Microstructure–property relationship in explosively welded duplex stainless steel–steel [J]. Materials Science and Engineering A, 2003, 363: 290-296.
[18] WRONKA B. Testing of explosive welding and welded joints. The microstructure of explosive welded joints and their mechanical properties [J]. Journal of Material Science, 2010, 45(13): 3465-3469.
[19] KAYA Y, KAHRAMAN N. An investigation into the explosive welding/ cladding of Grade A ship steel/AISI 316L austenitic stainless steel [J]. Materials and Design, 2013, 52(24): 367-372.
[20] KAHRAMAN N, GULENC B, FINDIK F. Joining of titanium/ stainless steel by explosive welding and effect on interface [J]. Journal of Materials Processing Technology, 2005, 169(2): 127-133.
[21] ATHAR M M H, TOLAMINEJAD B. Weldability window and the effect of interface morphology on the properties of Al/Cu/Al laminated composites fabricated by explosive welding [J]. Materials and Design, 2015, 86: 516-525.
[22] SARAVANAN S, RAGHUKANDAN K. Influence of interlayer in explosive cladding of dissimilar metals [J]. Materials and Manufacturing Processes, 2013, 28 (5): 589-594.
(Edited by FANG Jing-hua)
Cite this article as: Saravanan Somasundaram, Raghukandan Krishnamurthy, Hokamoto Kazuyuki. Effect of process parameters on microstructural and mechanical properties of Ti-SS 304L explosive cladding [J]. Journal of Central South University, 2017, 24(6): 1245-1251. DOI: 10.1007/s11771-017-3528-3.
Received date: 2016-03-23; Accepted date: 2016-09-30
Corresponding author: Saravanan Somasundaram, Assistant Professor, PhD; Tel: +91-9443676936; E-mail: ssvcdm@gmail.com, saravanan.s.04514@ annamalaiuniversity.ac.in