Trans. Nonferrous Met. Soc. China 23(2013) 120-127
Preparation and shape memory properties of TiO2/PLCL biodegradable polymer nanocomposites
Xi-li LU, Xiu-qian
, Jian-yong WANG, Zhi-jie SUN, Yun-xiang TONG
Center for Biomedical Materials and Engineering, Harbin Engineering University, Harbin 150001, China
Received 20 August 2012; accepted 15 October 2012
Abstract: The preparation of TiO2/poly(L-lactide-co-ε-caprolactone) (PLCL) nanocomposites and their properties were reported. TiO2 nanoparticles were surface modified by ring-opening polymerization of ε-caprolactone (ε-CL). The resulting poly(ε-caprolactone)-grafted TiO2 (g-TiO2) was characterized by Fourier transform infrared spectroscopy (FTIR), thermogravimetric analysis (TGA) and transmission electron microscopy (TEM). The g-TiO2 can be uniformly dispersed in chloroform and the g-TiO2/PLCL nanocomposites were successfully fabricated through solvent-casting method. The effects of the content of g-TiO2 nanoparticles on tensile properties and shape memory properties were investigated. A significant improvement in the tensile properties of the 5% g-TiO2/PLCL mass fraction nanocomposite is obtained: an increase of 113% in the tensile strength and an increase of 11% in the elongation at break over pure PLCL polymer. The g-TiO2/PLCL nanocomposites with a certain amount of g-TiO2 content have better shape memory properties than pure PLCL polymer. The g-TiO2 nanoparticles play an additional physical crosslinks which are contributed to improvement of the shape memory properties.
Key words: poly(L-lactide-co-ε-caprolactone); nanocomposites; TiO2 nanoparticles; surface grafting; shape memory properties; mechanical properties
1 Introduction
Thermal-responsive shape memory polymers (SMPs) are thermal-sensitive materials which can recover their original shape when they are heated to a temperature higher than a switching temperature. They have attracted an increasing attention in many fields, such as industry, aerospace field and biomedical field. For biomedical applications, the SMPs should be the biocompatible polymers and in many cases the SMPs also need to be biodegradable polymers. During the past years, biodegradable SMPs are of great importance in medical applications. Some successful applications, including intelligent sutures[1], smart cardiovascular implants [2] and drug release system [3], have been reported.
Poly(L-lactide) (PLLA) is a well-known biodegradable and biocompatible semi-crystalline polymer with a wide variety of biomedical applications [4,5]. Recently, it has been found that the PLLA has shape memory effect [6]. However, its high glass transition temperature and crystallinity lead to high shape recovery temperature and low shape recovery ratio. Copolymerization of L-lactide with ε-CL can be an appropriate method for the control of shape-memory behavior and other properties, thereby, widening the potential for biomedical applications of PLLA. In our previous report, the shape memory properties of PLCL copolymers with different compositions have been investigated systematically [7]. Although a relatively high recovery stress can be obtained in PLCL copolymer, the mechanical properties and recovery stress need to be enhanced to be suitable for use in various medical applications. The common method is to introduce the inorganic filler into polymer matrix to fabricate the composites. Various inorganic fillers such as hydroxyapatite, carbon nanotubes, Fe3O4 nanoparticles have been studied in previous reports on biodegradable shape memory composites in order to improve the mechanical properties or to derive the multiple functionalities [8-10]. Recently, TiO2 nanoparticles have been extensively studied due to their excellent mechanical properties, photocatalytic effects, as well as bioactive behavior [11-13]. In biomedical field, TiO2 nanoparticles are promising fillers used in biodegradable polymer matrices. Several authors have reported the effects of the addition of TiO2 nanoparticles on the mechanical properties and biodegradable properties [14-17]. However, to our knowledge, no reports about the effect of TiO2 nanoparticles on the shape memory properties can be found.
In this work, the nanocomposites based on PLCL and g-TiO2 nanoparticles were prepared. It is considered that the dispersion of nanofillers has a significant influence on the properties of nanocomposites. In order to improve the dispersion of the nanofiller, a novel approach was adopted to modify the TiO2 nanoparticles. The poly(ε-caprolactone) (PCL) was grafted onto the surface of TiO2 nanoparticles by ring-opening polymerization of ε-CL. The modified TiO2 nanoparticles were characterized by FTIR, TGA and TEM. The dependency of mechanical and shape memory properties of TiO2/PLCL nanocomposites on the content of g-TiO2 nanoparticles was investigated.
2 Experimental
2.1 Materials
ε-CL (99%, Aldrich) was distilled prior to use. TiO2 nanoparticles with an average size of 20 nm were purchased from Shanghai Huijinya Nanomaterials Company (China). The silane coupling agent NH2(CH2)3Si(OC2H5)3 (KH550) was purchased from Nanjing Jingtianwei Chemical Co., Ltd. PLCL copolymers were prepared in our own laboratory according to our previous work [7]. The relative molecular mass (Mr) of the PLCL was 210000, approximately. Stannous octanoate was obtained from Sigma and other agents were all of analytical grade and used as-received.
2.2 Preparation of surface-modified TiO2 nanoparticles
2.2.1 Pretreatment of TiO2 nanoparticles by silane coupling agent
Firstly, 3.2 g KH550 was dissolved in the mixture solution containing 160 mL ethnol and 7 mL deionized water by ultrasound for 30 min. Then 8.0 g TiO2 nanoparticles were added slowly into the mixture with stirring. And, the mixtures were ultrasound for additional 1 h. After that, the mixtures were stirred for 2 h at room temperature and 80 °C in water, respectively. Later, the pretreatment TiO2 (p-TiO2) nanoparticles were separated by centrifugation at 6000 r/min and washed with excessive amount of ethanol five times to completely remove the KH550. Finally, the p-TiO2 nanoparticles were dried in a vacuum oven at 60 °C for 24 h.
2.2.2 Grafting PCL onto surface of p-TiO2 nanoparticles
3 g p-TiO2 nanoparticles were dispersed in 100 mL toluene and 50 g freshly distilled ε-CL was slowly added under agitation. Stannous octanoate was then added as catalyst (the mole ratio of catalyst to ε-CL=1:2000) and the reaction mixture was stirred at 140 °C for 8 h under nitrogen protect. The reaction product was separated by centrifugation and washed with excessive amount of chloroform five times to remove the free PCL from the particles to obtain the surface-grafted TiO2 (g-TiO2). The g-TiO2 nanoparticles were dried in vacuum at 50 °C for 24 h to remove the residual chloroform.
2.3 Preparation of g-TiO2/PLCL nanocomposites
The g-TiO2/PLCL nanocomposites with 5%, 10% and 20% (mass fraction, the same below) g-TiO2 were prepared by solution casting method. The detailed procedure can be seen in our previous report [16].
2.4 Characterization
FTIR analysis was performed on a Perkin-Elmer 100 spectrophotometer. The samples were prepared by mixing the nanoparticles with KBr powders and pressing the mixture into disks. The samples of g-TiO2/PLCL nanocomposite films were examined by attenuated total reflectance (ATR) accessory.
Thermogravimetric analysis (TGA) was carried out on a thermogravimetric analyzer (Perkin-Elmer 7 series thermal analysis system) at a heating rate of 5 °C/min from room temperature to 700 °C under nitrogen atmosphere.
Transmission electron microscopy (TEM) investigations were taken using a Philips CM120 microscope at 200 kV acceleration voltages. The TEM specimens were prepared by dripping a drop of dispersed particle/CHCl3 suspension onto a TEM grid covered with carbon film and evaporating the solvent completely at room temperature.
2.5 Tensile properties
Tensile properties were determined by Instron 3365 electromechanical universal testing machine with a stretching rate of 10 mm/min. The dumbbell shaped samples with effective dimensions of 16 mm×4 mm×0.3 mm were cut from the nanocomposite films. All the experimental data were reported from the average values of three samples.
2.6 Shape memory behaviors
Shape memory behaviors of the nanocomposites were investigated by the tensile test. First, the sample was heated to 60 °C and then stretched to εm with a stretching rate of 10 mm/min. After that, the sample was cooled to 20 °C and maintained at this temperature for 5 min. Subsequently, the sample was unloaded and the retention strain at room temperature was εu. Finally, the stretched sample was heated again to 60 °C and the residual strain was εp. The shape retention rate (Rf) and the shape recovery rate (Rr) are defined as follows [7]:
(1)
(2)
For the measurement of the recovery stress, the stretched sample was clamped with its length fixed and then was heated to 60 °C and the tensile stress was recorded as the recovery stress.
3 Results and discussion
3.1 Characterization of g-TiO2 nanoparticles
Figure 1 shows the FTIR spectra of non-grafted TiO2, p-TiO2 and g-TiO2 nanoparticles. It can be seen from Fig. 1(a) that the absorption peak at 3430 cm-1 is ascribed to the vibration of hydroxyl group (—OH) at the surface of TiO2 nanoparticles. A broad absorption band between 500 and 1000 cm-1 is corresponding to the vibration absorption of the Ti—O—Ti linkages for TiO2 nanoparticles [12]. Because the TiO2 nanoparticles surface can absorb water in the air, the absorption peak at 1630 cm-1 is attributed to the vibration of hydrogen bond. From Fig. 1(b), it is clearly observed that the intensities of the absorption peaks at 3430 and 1630 cm-1 are relatively strong compared with the non-grafted TiO2, which may be attributed to the interaction of hydroxyl group of the surface of TiO2 nanoparticles with the KH550. In addition, the absorption peaks at 2930 and 2850 cm-1 are assigned to the stretching vibration of C—H and (—CH2—) in KH550, respectively. In Fig. 1(c), a new carbonyl stretching band at 1745 cm-1 occurs, which indicates that the PCL polymer chains have been successfully grafted onto the surface of TiO2 nanoparticles.
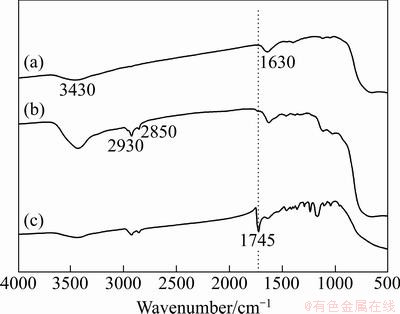
Fig. 1 FTIR spectra of non-grafted TiO2 (a), p-TiO2 (b) and g-TiO2 (c) nanoparticles
TGA is used to evaluate the content of the PCL polymer chains grafted onto the surface of TiO2 nanoparticles. Figure 2 shows the TGA curves of non-grafted TiO2 and g-TiO2 nanoparticles. It can be seen that non-grafted TiO2 nanoparticles display a little mass loss and the total mass loss of non-grfted TiO2 nanoparticles is about 4.0% when heated from room temperature to 700 °C. However, the g-TiO2 nanoparticles show appreciable mass loss from 200 to 600 °C, which is attributed to the decomposition of the grafted PCL polymer chains. Thus, the amount of PCL polymer chains grafted on the TiO2 nanoparticles surface was calculated as R=Δm1-Δm2, where R is the grafting ratio, Δm1 is mass loss rate of g-TiO2 and Δm2 is mass loss rate of non-grafted TiO2. The result shows that the grafting ratio of g-TiO2 nanoparticles is 19.4%.
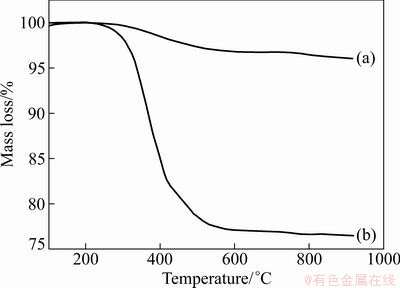
Fig. 2 TGA curves of non-grafted TiO2 (a) and g-TiO2 (b) nanoparticles
The TEM images of TiO2 and g-TiO2 nanoparticles dispersed in chloroform are shown in Fig. 3. It can be seen from Fig. 3(a) that the non-grafted TiO2 nanoparticles aggregate seriously and cannot be distinguished each other. However, in Fig. 3(b), the g-TiO2 nanoparticles display obviously improved dispersibility, which can be attributed to the PCL polymer chains surrounding on the surface of TiO2 nanoparticles.
For purpose of assessing nanoparticles dispersion, the non-grafted TiO2 and g-TiO2 nanoparticles are dispersed in chloroform with the same concentration of 5 g/L to prepare the suspension. The photographs of the dispersed suspension holding for 24 h are shown in Fig. 4. It can be seen that the suspension with the g-TiO2 nanoparticles exhibits homogeneous mixed solution after holding for 24 h (Fig. 4, right). However, the suspension with the non-grafted TiO2 nanoparticles is almost transparent and the large number of non-grafted TiO2 nanoparticles stay on the bottom of the bottle (Fig. 4, left), which shows that the non-grafted TiO2 nanoparticles cannot be well dispersed in chloroform.
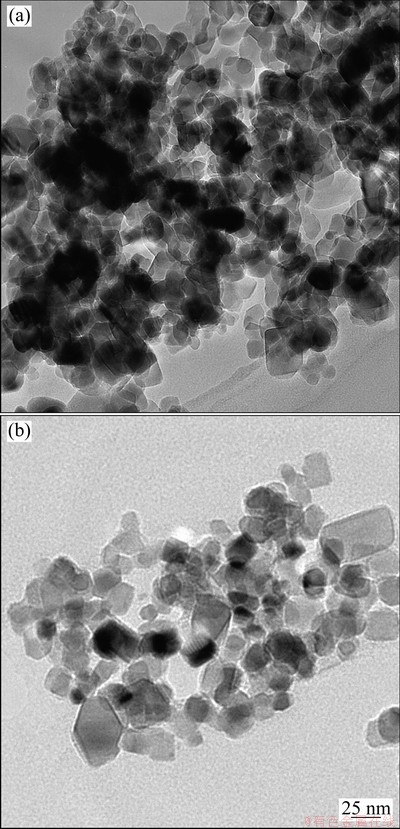
Fig. 3 TEM images of non-grafted TiO2 (a) and g-TiO2 (b) nanoparticles dispersed in chloroform
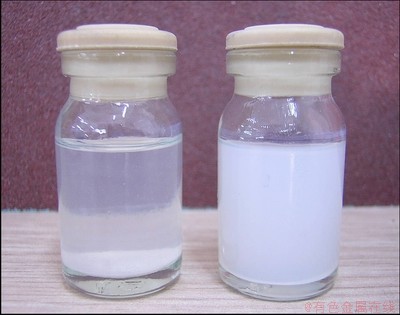
Fig. 4 Photographs of suspension for non-grafted TiO2 (left) and g-TiO2 nanoparticles dispersed in chloroform after holding for 24 h
3.2 Characterization of g-TiO2/PLCL nanocomposite
FTIR spectra of the nanocomposites were measured to evaluate the correlation between the g-TiO2 nanoparticles and the PLCL matrix. The FTIR spectra of pure PLCL and g-TiO2/PLCL nanocomposites with different g-TiO2 contents are exhibited in Fig. 5. It can be seen that the characteristic peaks of pure PLCL are observed in the FTIR spectra of g-TiO2/PLCL nanocomposites. There are no apparent changes in the characteristic absorption peaks, which indicates that there is almost no chemical reaction between the g-TiO2 nanoparticles and the PLCL matrix.
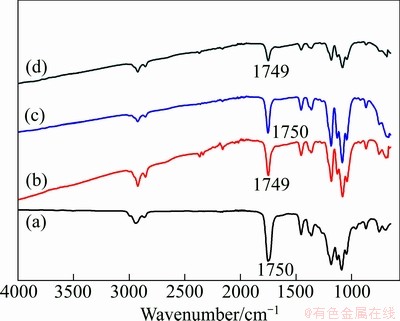
Fig. 5 FTIR spectra of pure PLCL (a), 5% g-TiO2/PLCL (b), 10% g-TiO2/PLCL (c) and 20% g-TiO2/PLCL (d) nano- composites
The DSC curves of pure PLCL and g-TiO2/PLCL nanocomposites are shown in Fig. 6. From these curves, no endothermic peaks can be observed, which means that the pure PLCL and PLCL in the composites are amorphous. The glass transition temperature (Tg) of the pure PLCL and the nanocomposites with 5%, 10% and 20% g-TiO2 nanoparticles are 45.4, 44.6, 43.2 and 42.5 °C, respectively. It is seen that the Tg of the nanocomposites slightly decreases with the addition of g-TiO2 nanoparticles. The g-TiO2 nanoparticles may transfer the heat during the glass transition of the polymer, which leads to the change of Tg.
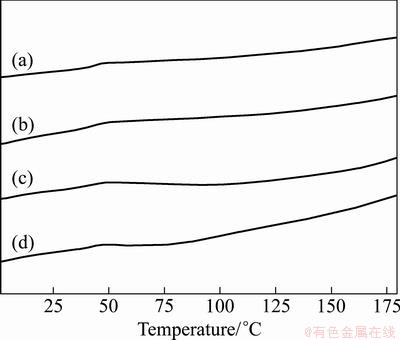
Fig. 6 DSC curves of pure PLCL (a), 5% g-TiO2/PLCL (b), 10% g-TiO2/PLCL (c) and 20% g-TiO2/PLCL (d) nanocomposites
3.3 Tensile properties of g-TiO2/PLCL nanocomposite
Figure 7(a) shows the stress—strain curves of the samples of pure PLCL and g-TiO2/PLCL nanocomposites at room temperature. It is seen that the yielding phenomenon is observed in all specimens. The tensile properties of the samples are summarized in Fig. 7 (b). It is observed that the ultimate tensile strength and the elongation at break of the nanocomposites increase with an increase of the g-TiO2 content and possess the maximum value of 5% for the g-TiO2 loading. With further increasing the g-TiO2 content, the ultimate tensile strength and the elongation at break of the nanocomposites start to decrease. For 5% g-TiO2 nanocomposites, the ultimate tensile strength and the elongation at break increase to 35.4 MPa and 444.6%, which are 113% and 11% higher than that of pure PLCL, respectively.
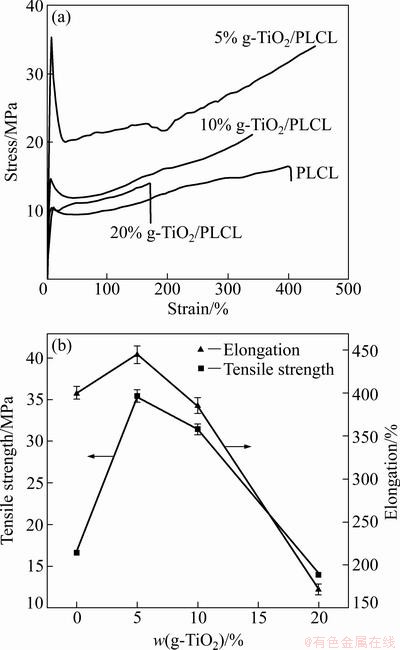
Fig. 7 Stress—strain curves of pure PLCL and g-TiO2/PLCL nanocomposites with different compositions (a) and effect of g-TiO2 content on tensile strength and elongation at break (b)
It is recognized that the tensile properties of nanocomposites mainly depend on the degree of dispersion and the adhesion between the filler and the matrix [11]. Under a lower nanoparticles loading, the dispersion of g-TiO2 nanoparticles in PLCL matrix is fairly uniform, thus the g-TiO2 nanoparticles exhibit the nanoreinforcing effect due to their large specific surface area. In addition, even though there is no chemical bonding between g-TiO2 nanoparticles and PLCL matrix, the adhesion between the g-TiO2 nanoparticles and PLCL matrix is still enhanced because the PCL polymer chains grafted onto the surface of TiO2 nanoparticles can penetrate into the PLCL matrix and entangle with the PLCL chains. Therefore, the load can be transferred more effectively between the particles and the matrix. This can explain the improvement of tensile properties with a small amount of g-TiO2 nanoparticles. Under a higher nanoparticles loading, the g-TiO2 nanoparticles have a tendency to agglomerate in the PLCL matrix. The agglomerated nanoparticles act as stress concentration causing the decrease in the tensile strength. Moreover, the mobility of PLCL chain can be obstructed by the agglomerated nanoparticles, as a result the elongation at break of the nanocomposites decreases.
3.4 Shape memory properties of g-TiO2/PLCL nanocomposite
In order to demonstrate the shape memory property, the recovery process of a circular specimen of the 5% g-TiO2/PLCL was investigated. A circular specimen was heated at 60 °C and gave a temporary folded shape by bending and then cooled to fix the shape. When the sample is heated again, it starts to recover its original shape which is photographed at different times, as shown in Fig. 8. Figure 8 clearly shows the shape memory composites with almost a completely shape recovery.
Figure 9(a) shows the effects of the content of g-TiO2 nanoparticles on the shape recovery rate and shape retention rate of the nanocomposites at a prestrain of 100%. It is seen that pure PLCL and g-TiO2/PLCL nanocomposites show both good shape recovery and shape fixing performance. The shape recovery rate and shape retention rate of all samples exceed 90%. Furthermore, by comparing with the pure PLCL, the shape recovery rate and shape retention rate of the g-TiO2/PLCL nanocomposites are improved even the g-TiO2 content is as high as 10%. The improvement of shape memory properties of the nanocomposites is ascribed to the addition of g-TiO2 nanoparticles. Regarding the mechanism of shape memory effect for polymer, the polymer must include the reversible phase and the fixed phase. According to the DSC results mentioned in section 3.1, the PLCL and the g-TiO2/PLCL nanocomposites are amorphous. Therefore, the entanglements of molecular chain are expected to serve as the fixing phase, while the flexible amorphous PLCL polymer is selected as a reversible phase. However, for g-TiO2/PLCL nanocomposites, an appropriate amount of g-TiO2 nanoparticles play a positive role in shape memory. First, the g-TiO2 nanoparticles obstruct the movement of PLCL molecular chains, which contributes to the shape fixing. Second, the PCL polymer chains grafted onto the surface of TiO2 nanoparticles can mix and entangle with the PLCL matrix chains, thus the g-TiO2 nanoparticles act as physical crosslink points that prevent the relative slippage between the polymer chains. However, if there are too many g-TiO2 nanoparticles, the movements of chain segments of amorphous PLCL polymer are greatly restricted during shape recovery, which results in the decrease of shape recovery performance.
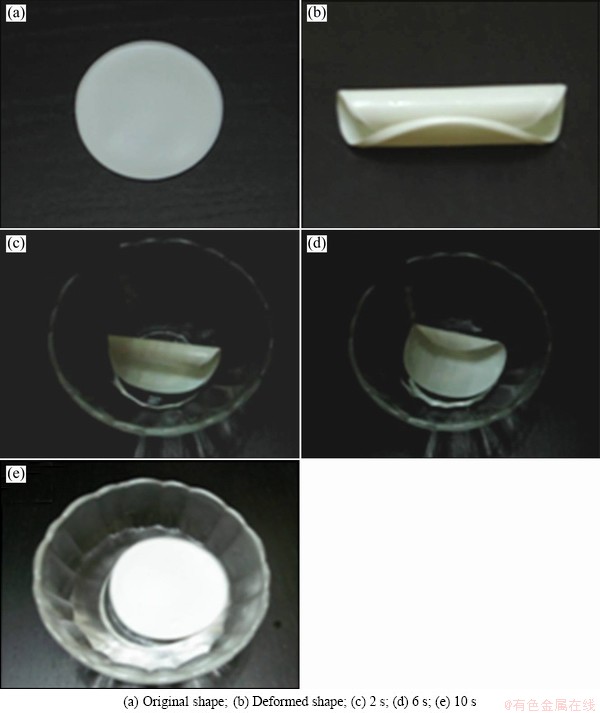
Fig. 8 Shape recovery process of circular shape of 5% g-TiO2/PLCL nanocomposites at 60 °C at different times
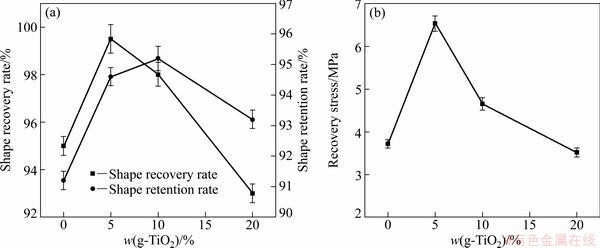
Fig. 9 Effect of content of g-TiO2 nanoparticles on shape recovery rate and shape retention rate (a) and recovery stress of nanocomposites (prestrain=100%) (b)
The effect of the content of g-TiO2 nanoparticles on the recovery stress of the nanocomposites at a prestrain of 100% is shown in Fig. 9(b). It is noted that the recovery stress initially increases with the increase of g-TiO2 content and then decreases as g-TiO2 content increases further. The highest recovery stress of 6.5 MPa is seen for the 5% g-TiO2 nanocomposite. This value is 43% higher than that of pure PLCL.
The increase of the recovery stress is thought to be related to the mechanical reinforcement effects of the filler. As mentioned in section 3.2, the tensile test results show that the addition of g-TiO2 enhances the tensile strength. Thus, the generated stress during the motion of polymer chains will increase, which may cause a rise in recovery stress of nanocomposites. Moreover, according to the rubber elasticity theory, the recovery stress is proportional to the number of crosslinks existing in the polymeric matrix. The addition of g-TiO2 nanoparticles provides the additional physical crosslinks, which can cause the recovery stress to increase. Additional physical crosslinks can be formed by the entanglements between the PCL polymer chains grafted onto the surface of TiO2 and the chains of PLCL polymer matrix. An appropriate amount of g-TiO2 can make a good distributed system, thus the possibility of entanglements mentioned above is high.
4 Conclusions
1) PCL polymer chains were successfully grafted onto the surface of the TiO2 nanoparticles by ring- opening polymerization of ε-CL. The g-TiO2 nanoparticles have a better distribution in chloroform than the non-grafted TiO2 nanoparticles. Thus, the uniformed g-TiO2/PLCL biodegradable shape memory nanocomposites were prepared.
2) The introduction of the g-TiO2 nanoparticles into PLCL matrix can improve the mechanical properties. The g-TiO2/PLCL nanocomposites have excellent shape memory properties. When the content of g-TiO2 nanoparticles is 5%, the nanocomposite possesses the highest shape recovery rate and the recovery stress. It is found that the g-TiO2 nanoparticles play an important role in enhancement of mechanical properties and shape memory properties.
3) These g-TiO2/PLCL nanocomposites have a potential for application in minimally invasive surgery due to their good mechanical properties, excellent shape memory properties as well as bioactive behavior of TiO2 nanoparticles.
References
[1] LENDLEIN A, LANGER R. Biodegradable, elastic shape-memory polymers for potential biomedical applications [J]. Science, 2002, 296: 673-676.
[2] XUE L, DAI S Y, LI Z. Biodegradable shape-memory block co-polymers for fast self-expandable stents [J]. Biomaterials, 2010, 31: 8132-8140.
[3] CONCEPCION SERRANO M, CARBAJAL L, AMEER G A. Shape-memory polymers: Novel biodegradable shape-memory elastomers with drug-releasing capabilities [J]. Adv Mater, 2011, 23: 2211-2215.
[4] FENG K, SUN H, BRADLEY M A, DUPLERE J, GIANNOBILE W V, MA P X. Novel antibacterial nanofibrous PLLA scaffolds [J]. J Control Release, 2010, 146: 363-369.
[5] TIAN H, TANG Z, ZHUANG X, CHEN X, JING X. Biodegradable synthetic polymers: Preparation, functionalization and biomedical application [J]. Prog Polym Sci, 2012, 37: 237-280.
[6] WONG Y S, VENKATRAMAN S S. Recovery as a measure of oriented crystalline structure in poly(L-lactide) used as shape memory polymer [J]. Acta Mater, 2010, 58: 49-58.
[7] LU X L, CAI W, GAO Z Y. Shape-memory behaviors of biodegradable poly(L-lactide-co-ε-caprolactone) copolymers [J]. J Appl Polym Sci, 2008, 108: 1109-1115.
[8] ZHENG X T, ZHOU S B, LI H, WENG J. Shape memory properties of poly(D,L- lactide)/hydroxyapatite composites [J]. Biomaterials, 2006, 27: 4288-4295.
[9] FENG J T, CAI W, SUI J H, CHAKOLI A. Poly(l-lactide) brushes on magnetic multiwalled carbon nanotubes by in-situ ring-opening polymerization [J]. Polymer, 2008, 49: 4989-4994.
[10] ZHENG X T, ZHOU S B, XIAO Y, YU X J, LI X H, WU P Z. Shape memory effect of poly(d,l-lactide)/Fe3O4 nanocomposites by inductive heating of magnetite particles [J]. Colloids Surf. B: Biointerfaces, 2009, 71: 67-72.
[11] TAO Jie, DENG Jie, DONG Xiang, ZHU Hong, TAO Hai-jun. Enhanced photocatalytic properties of hierarchical nanostructured TiO2 spheres synthesized with titanium powders [J]. Transactions of Nonferrous Metals Society of China, 2012, 22(8): 2049-2056.
[12] NAKAYAMA N, HAYASHI T. Preparation and characterization of poly(l-lactic acid)/TiO2 nanoparticle nanocomposite films with high transparency and efficient photodegradability [J]. Polym Degrad Stab, 2007, 92: 1255-1264.
[13] FUKUDA N, TSUJI H. Physical properties and enzymatic hydrolysis of poly(L-lactide)/TiO2 composites [J]. J Appl Polym Sci, 2005, 96: 190-199.
[14] BOCCACCINI A R, GERHARDT L C, REBELING S, BLAKER J J. Fabrication, characterisation and assessment of bioactivity of poly(d,l-lactid acid) (PDLLA)/TiO2 nanocomposite films [J]. Composite A, 2005, 36: 721-727.
[15] LUO Y B, WANG X L, WANG Y Z. Effect of TiO2 nanoparticles on the long-term hydrolytic degradation behavior of PLA [J]. Polym Degrad Stab, 2012, 97: 721-728.
[16] LU X L, LV X Q, SUN Z J, ZHENG Y F. Nanocomposites of poly(L-lactide) and surface-grafted TiO2 nanoparticles: Synthesis and characterization [J]. Eur Polym J, 2008, 44: 2476-2481.
[17] LUO Y B, LI W D, WANG X L, XU D Y, WANGY Z. Preparation and properties of nanocomposites based on poly(lactic acid) and functionalized TiO2 [J]. Acta Mater, 2009, 57: 3182-3191.
TiO2/PLCL可降解聚合物纳米复合材料的制备及形状记忆性能
鲁玺丽,吕秀乾,王建永,孙志洁,佟运祥
哈尔滨工程大学 生物医学材料与工程研究中心,哈尔滨 150001
摘 要:制备TiO2/聚(L-丙交酯-ε-己内酯) (PLCL)纳米复合材料并研究其性能。采用ε-己内酯开环聚合法对TiO2纳米粒子进行表面改性,通过傅立叶变换红外光谱(FTIR)、热重分析(TGA)和透射电子显微分析(TEM)对聚己内酯(PCL)接枝改性后的TiO2纳米粒子(g-TiO2)进行表征。g-TiO2纳米粒子能均匀地分散在三氯甲烷溶液中。采用溶液浇铸的方法成功地制备了TiO2/PLCL复合材料。研究g-TiO2纳米粒子的含量对材料力学性能和形状记忆性能的影响。结果表明,5% g-TiO2/PLCL复合材料的力学性能有显著的提高,与纯PLCL相比,抗拉强度提高了113%,伸长率提高了11%。含有g-TiO2纳米粒子的复合材料的形状记忆性能优于纯PLCL。g-TiO2纳米粒子具有物理交联作用,有助于形状记忆效应的提高。
关键词:聚(L-丙交酯-ε-己内酯);纳米复合材料;TiO2纳米粒子;表面接枝;形状记忆性能;力学性能
(Edited by Xiang-qun LI)
Foundation item: Project (50903023) supported by the National Natural Science Foundation of China; Project (HEUCF201210005) supported by the Fundamental Research Funds for the Central Universities, China; Project (2010RFQXG037) supported by Harbin Special Fund for Innovation Talents of Science and Technology, China
Corresponding author: Xi-li LU; Tel: +86-451-82518173; E-mail: lusissi1975@126.com
DOI: 10.1016/S1003-6326(13)62437-1