
Preparation of TiC powders by carbothermal reduction method in vacuum
SEN Wei1, 2, 3, XU Bao-qiang1, 2, YANG Bin1, 2, SUN Hong-yan1, 2,
SONG Jian-xun1, 2, WAN He-li1, 2, DAI Yong-nian1, 2
1. National Engineering Laboratory for Vacuum Metallurgy, Kunming 650093, China;
2. Key Laboratory of Vacuum Metallurgy for Nonferrous Metal of Yunnan Province, Kunming 650093, China;
3. Faculty of Materials Science and Engineering, Kunming University of Science and Technology,
Kunming 650093, China
Received 28 January 2010; accepted 4 May 2010
Abstract: The preparation of fine TiC powders by carbothermal reduction of TiO2 in vacuum was investigated by XRD, SEM, XRF and laser particle sizer. Thermodynamic analysis indicates that it is easy to prepare TiC in vacuum and the formation sequence of products are Ti4O7 (Magneli phase), Ti3O5, Ti2O3, TiCxO1-x and TiC with the increase of reaction temperature. Experimental results demonstrate that TiC powders with single phase are obtained with molar ratio of TiO2 to C ranging from 1:3.2 to 1:6 at 1 550 °C for 4 h when the system pressure is 50 Pa, and TiC1.0 is gained when the molar ratio of TiO2 to C is 1:4 and 1:5. In addition, fine TiC1.0 powders (D50 equals 3.04 μm) with single phase and low impurities are obtained when the molar ratio of TiO2 to C is 1:4. SEM observation shows that uniform shape, low agglomeration, and loose structure are observed on the surface of block product.
Key wards: titanium dioxide; titanium carbide; vacuum; carbothermal reduction method
1 Introduction
Titanium carbide, with cubic crystal structure of NaCl type, has been widely used in many fields, such as aerospace materials, cutting tools, composite ceramic materials and wear resistance tools[1], because of its outstanding properties, including high melting temperature (3 067 °C), high elastic modulus (410-450 GPa), high Vickers hardness (29-34 GPa), high electrical conductivity (30×106 S/cm) and high solvency with other carbides[2-3].
The main methods to synthesize TiC powders are as follows: 1) carbothermal reduction of TiO2 powders using carbon[4-5] or carbonaceous organic material [6-7]; 2) carbothermal reduction of polymeric precursors obtained from titanium alkoxides and other organic compounds in inert atmosphere[8]; 3) direct reaction between metallic titanium and carbon by self propagation high-temperature synthesis(SHS) or mechanical ball milling [9-11]; (4) gas phase reaction of TiCl4 and gaseous hydrocarbons[12]. Among them the method of carbothermal reduction of TiO2 powders is one of the appropriate routes to produce TiC powders for its inexpensive raw materials and simple process. According to the result of WEIMER[13], the synthesis of TiC by this method requires high temperature (1 700-2 100 °C) and long reaction time (10-24 h) in argon atmosphere. So it presents the problems of particle agglomeration, grain growth, non-uniform particle shape and considerable quantities of unreacted titanium dioxide and carbon in the product[3]. Therefore, for resolving these problems the formation of TiC powders by carbothermal reduction in vacuum was researched. AFIR et al[14] investigated the Ti-O-C system in a continuous vacuum. In their study the reduction of TiO2 by graphite or metallic titanium was monitored in an oven for X-rays observation with a graphite resistance under continuous vacuum. They determined the crystalline characteristics at high temperature for all observed solid phases as well as their composition: TiO2-x, TinO2n-1 with 9≥n≥4, Ti2O3, TiO, Ti2O, TiC1-xOx, and TiC.
In previous study, the possibility of preparing TiC by using carbothermal reduction of TiO2 in vacuum was investigated, and the trace amounts of TiC in product were observed[15]. The main purpose of this work is to produce homogeneous and fine TiC powders through carbothermal reduction in vacuum. For optimizing the process parameters, the effects of different reaction temperature and molar ratio of TiO2 to C on reaction products are also studied based on thermodynamic analysis.
2 Experimental
Titania (TiO2≥98.6%, mass fraction) was used as raw material and charcoal (C≥89%, mass fraction) as reductant in experiments. The average particle size of TiO2 is 0.1-0.15 μm.
For preparing TiC powders, the mixtures of titania and charcoal with certain molar ratio were milled 6-10 h in planetary-type ball milling at the rotating speed of 100-400 r/min. The mixed powders were compressed into blocks by uni-axial pressing in a hardened steel die under 4-6 MPa. Then the blocks were removed into crucibles and put into vacuum furnace. The feed was heated to different reaction temperatures at a heating rate of 10 °C/min and the temperature was held for 4 h when the system pressure was 50 Pa. Then the vacuum furnace power was switched off when products were cooled down to 200 °C. The reduced products were obtained, and fine TiC powders were gained via crushing and milling.
Lattice parameter and phase composition of the prepared TiC were investigated by X-ray diffraction instrument (D/max-3B) using Cu Kα radiation in the range of 10°-95° (2θ) with a step of 5(°)/min. The morphology, element content and grain size distribution were studied by scanning electron microscopy (SEM, EPMA-8705), X-ray fluorescence spectrophotometer (XRF, ZSX100e) and laser particle sizer (LPS, LS800), respectively.
3 Results and discussion
3.1 Thermodynamic analysis
According to the data and formula in Ref.[16], the Gibbs free energy and reaction enthalpy for the carbothermal reduction of TiO2 can be calculated. So the initial reaction temperature at different pressures could be obtained. Some potential reactions between TiO2 and C are listed in Table 1, and the following reactions are included in its reaction (b)-(d):
3Ti4O7+C=4Ti3O5+CO (1)
2Ti3O5+C=3Ti2O3+CO (2)
(1/2)Ti3O5+C=(3/2)TiO+CO (3)
Table 1 Reactions for TiO2-C system
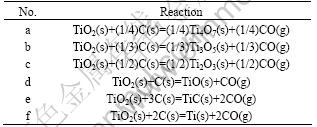
The relationship between reaction enthalpy and temperature is shown in Fig.1, which illustrates that the order of reaction heat from reaction (a) to reaction (f) increase gradually. It indicates that reactions can conduct only when heat is enough. Therefore, higher temperature and longer holding time are necessary for preparing large amounts of TiC.
The Gibbs free energy of reaction (e) at different pressures is shown in Fig.2, which is based on the initial reaction temperatures of 1 271, 848, 787, 711, 645 and 588 °C when the system pressures are 105, 50, 10, 1, 0.1 and 0.01 Pa Pa, respectively. It is apparent that the initial
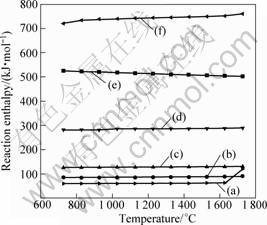
Fig.1 Reaction enthalpy of reactions (a)-(f) as function of temperature
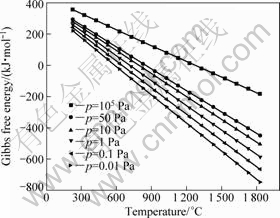
Fig.2 Gibbs free energy of reaction (e) at different pressure (p) as function of temperature
reaction temperature of reaction (e) decreases obviously when the system pressure declines. Therefore, the preparation of TiC in vacuum is easier than that at atmospheric pressure. What’s more, large amounts of TiC form easily for the gas CO escapes fast from raw material (TiO2 and C) in vacuum.
3.2 Effects of reaction temperature on reaction products
The formation of phase in carbothermal process was studied in temperature range of 1 100 °C-1 550 °C. Figure 3 shows XRD patterns of products prepared at 1 (0) as function of temperature 100, 1 200, 1 300, 1 400, 1 500 and 1 550 °C for 4 h when the molar ratio of TiO2 to C is 1:4. It can be seen that Ti3O5 phase and Magneli phase (Ti4O7) appear at 1 100 °C. At 1 200 °C, a large amount of Ti2O3 phases form while the peak intensity of Ti3O5 diminishes and Ti4O7 disappears. At the same time, trace amount of solid solution TiCxO1-x is formed easily from TiC and TiO because their lattice parameters are approximately the same[1,14]. At 1 300 °C, TiC phase appears and the peak intensities of solid solution TiCxO1-x increase when the peaks of Ti2O3 diminish and Ti3O5 disappears, which indicates that TiC phase may form from TiCxO1-x with further reduction. At 1 400 °C and 1 500 °C, Ti2O3 peak intensities diminish gradually and TiCxO1-x disappear while TiC peak intensities increase. At 1 550 °C, only TiC phase is formed. The results illustrate that the formation sequence of the products in vacuum should be Magneli phase (Ti4O7), Ti3O5, Ti2O3, TiCxO1-x and TiC.
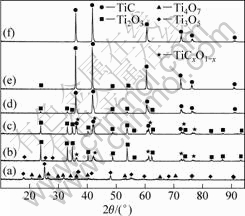
Fig.3 XRD patterns of products prepared at different temperatures: (a) 1 100 °C; (b) 1 200 °C; (c) 1 300°C; (d) 1 400 °C; (e) 1 500 °C; (f) 1 550 °C
Furthermore, Gibbs free energy of reactions (a)-(f) at 50 Pa was calculated and shown in Fig.4, which is based on the initial reaction temperatures of reactions (a)-(f) at 666, 709, 761, 926, 848 and 1 209 °C, respectively. The results indicate that the formation sequence of products should be Ti4O7, Ti3O5, Ti2O3, TiC, TiO and Ti with increasing reaction temperature. But Ti phase is not detected in experiments owing to the fact that reaction (f) needs more reaction heat (Fig.1) and its Gibbs free energy is higher than that of reaction (e). On the contrary, TiC can be prepared easily because the Gibbs free energy of reaction (e) is the lowest at higher temperature.
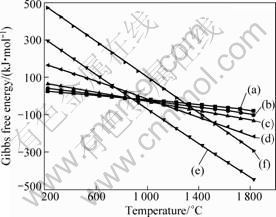
Fig.4 Gibbs free energy of reaction (a)-(f) as function of temperature at 50 Pa
The analysis from Fig.3 also implies that Ti2O3 is the lowest oxide phase before forming TiCxO1-x and TiC when carbon content in raw materials is enough. This is inconsistent with other work concerning Ti3O5 [17-18] and TiO[19] as lowest oxide of the system, while consistent with the observation of KOC[3] and BERGER[4].
In fact, carbothermal reduction reaction of TiO2 is a continuous process. Based on XRD results in Fig.3 and reaction thermodynamics between TiO2 and C, the main reactions are:
nTiO2+C=TinO2n-1+CO (n>4) (4)
4TinO2n-1+(n-4)C=nTi4O7+(n-4)CO (n>4) (5)
3Ti4O7+C=4Ti3O5+CO (6)
2Ti3O5+C=3Ti2O3+CO (7)
Ti2O3+(1+4x)C=2TiCxO1-x+(1+2x)CO (8)
TiCxO1-x+(2-2x)C=TiC+(1-x)CO (9)
3.3 Effects of different molar ratio of TiO2 to C on reaction products
Figure 5 shows XRD patterns of the reaction products prepared at 1 550 °C for 4 h when the molar ratio of TiO2 to C ranges from 1:1 to 1:6. As seen in patter (a), Ti2O3 and TiO phase appear when the molar ratio of TiO2 to C is 1:1. In pattern (b), the solid solution TiCxO1-x is formed when Ti2O3 peak intensities diminish and TiO disappears. In pattern (c), TiC phase is formed when Ti2O3 peak intensities decrease and TiCxO1-x disappears. The results indicate that TiC phase may be formed at the expense of titanium oxides and oxycarbides when the molar ratio of TiO2 to C decreases. Therefore, only TiC phase is observed when the molar ratio of TiO2 to C decreases continually (pattern (d)-(g)). But excessive carbon is observed in TiC powders with the molar ratio of 1:4, 1:5 and 1:6 when dipped in acid. The excessive carbon is not detected in patterns (e)-(g) since the charcoal powders are amorphous carbon and small amounts of them cannot be detected by XRD instrument. However, the excessive carbon may be separated from TiC powders by combustion method. Therefore, pure TiC powders will be gained in molar ratio range of 1:3.2 to 1:6.
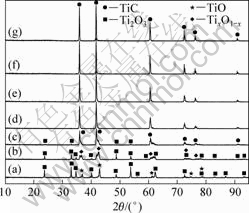
Fig.5 XRD patterns of reaction products prepared at 1 550 °C with different molar ratio of TiO2 to C: (a) 1:1; (b) 1:2; (c) 1:3; (d) 1:3.2; (e) 1:4; (f) 1:5; (g) 1:6
The finer the stoichiometric TiC powder particles, the higher the mechanical strength of the consolidated materials obtained. So it is necessary to calculate the lattice parameter for defining the stoichiometry of TiC powders by means of extrapolation technique[20-23] with the following equation:
(10)
(11)
where θ is the diffraction angle, a is the lattice parameter which could be determined by Eq.(11). a0 is the true lattice parameter of TiC which could be determined by Eq.(10). In accordance with XRD patterns, we can draw the figure of lattice parameter versus Nelson-Riley function F(θ) and extrapolate to a0 as θ=90°.
The calculated Nelson-Riley extrapolation plots for TiC powders prepared at 1 550 °C for 4 h when the molar ratio of TiO2 to C is 1:3, 1:3.2, 1:4, 1:5, respectively are shown in Fig.6. The corresponding lattice parameters extrapolated using this method are 0.431 45, 0.431 60, 0.432 40 and 0.432 40 nm, respectively. According to the International Centre for Diffraction Data (JCPDS-ICDD 2000) Powder Diffraction File (PDF) card 65-0242, the relevant lattice parameters of TiC is 0.432 7 nm. So the calculated results indicate that the extrapolated values deviate from the standard value and become close to it when the molar ratio of TiO2 to C decrease, which can be attributed to the reasons as follows. First, the strain and lattice defect are induced by changing reaction temperature. Second, sub-stoichiometric composition exists in the prepared TiC powders for TiC exists over a very wide compositional range according to Ti-C phase diagram[24]. The atom ratio of C to Ti varies from 0.49 to 0.95. Therefore, sub-stoichiometric TiCx (x<1) may exists in TiC powders. However, we may accept TiC powders as TiC1.0 when the extrapolated values with molar ratio of 1:4 and 1:5 are slightly inferior to standard value.
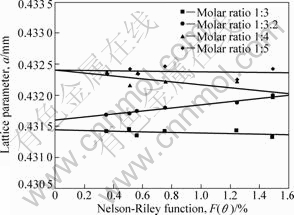
Fig.6 Nelson-Riley extrapolation plots for TiC powders prepared at 1 550 °C for 4 h with different molar ratio
3.4 Characterization of fine TiC powders prepared at 1 550 °C for 4 h
From the above results, TiC powders that are near stoichiometry were prepared at 1 550 °C for 4 h when the molar ratio of TiO2 to C is 1:4. Figure 7 shows SEM micrographs of the surface of TiC block. From Figs.7(a)-(c), we can see that the morphology of the surface of TiC block is homogeneous and loose agglomeration. It can be seen from Figs.7(a) and (d) that among TiC crystal grains lots of gas porosities distribute, which are caused by discharged gas CO in carbothermal reduction process. The results illustrate that the increasing contact area between reactants by ball milling should result in a more complete reaction and uniform shape. In addition, loose structure can be formed owing to the gas CO escaping easily in vacuum. Consequently,
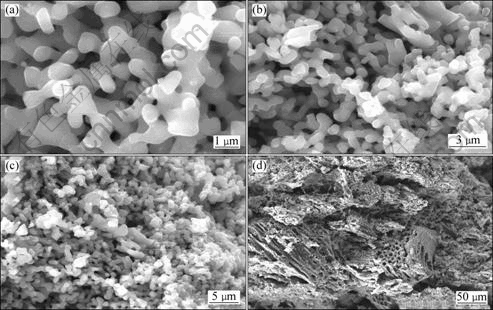
Fig.7 SEM micrographs of surface of TiC block prepared at 1 550 °C
fine TiC powders were gained via crushing and milling. Laser particle analysis shows that the grain size ranges from 1.13 μm to 5.69 μm (D10-D90) while D50 and specific surface area are 3.04 μm, 2.96 m2/cm3, respectively.
Fig.8 shows XRD pattern of ultrafine TiC powders prepared at 1 550 °C with molar ratio of 1:4. The XRD analysis for the product shows the clean peak of the fine TiC powders. Furthermore, XRF (X-ray fluoroscopy) analysis in Table 2 reveals that the content of impurities is very low in the fine TiC powders.
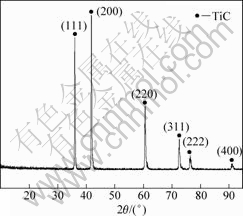
Fig.8 XRD pattern of ultrafine TiC powders prepared at 1 550 °C for 4 h (molar ratio 1:4)
Table 2 Content of impurities by XRF analysis (mass fraction, %)

4 Conclusions
1) Based on XRD and thermodynamic analyses, the formation sequence of TiC powders prepared in vacuum is Magneli phase (Ti4O7), Ti3O5, Ti2O3, TiCxO1-x and TiC with temperature increasing. Moreover, the preparation of TiC in vacuum is easier than at atmosphere pressure, and large amounts of TiC form easily in vacuum.
2) TiC powders with single phase were obtained on conditions that the molar ratio of TiO2 to C ranges from 1:3.2 to 1:6 at 1 550 °C for 4 h when the system pressure is 50 Pa. Lattice parameter calculation indicates that TiC1.0 should exist in TiC powders when the molar ratio of TiO2 to C is 1:4 and 1:5.
3) Fine TiC (D50 equals 3.04 μm) powders with low impurities can be prepared by ball milling and heating the mixture of titania and charcoal (1:4) at 1 550 °C for 4 h under the system pressure of 50 Pa. SEM micrograph shows homogeneous morphology, low agglomeration and loose structure on the surface of block.
References
[1] MO Wei. Titanium metallurgy [M]. Beijing: Metallurgical Industry Press, 1998: 68-70. (in Chinese)
[2] GOTOH Y, FUJIMURA K, KOIKE M, OHKOSHI Y, NAGURA M, AKAMATSU K, DEKI S. Synthesis of titanium carbide from a composite of TiO2 nanoparticles/methyl cellulose by carbothermal reduction [J]. Materials Research Bulletin, 2001, 36(13/14): 2263-2275.
[3] KOC R. Kinetic and phase evolution during carbothermal synthesis of titanium carbide from ultrafine titania/carbon mixture [J]. Journal of Materials Science, 1998, 33(4): 1049-1055.
[4] BERGER L M. Titanium carbide synthesis from titanium dioxide and carbon black [J]. International Journal of Refractory Metals and Hard Materials, 1992, 3(1): 3-15.
[5] MAITRE A, TETARD D, LEFORT P. Role of some technological parameters during carburizing titanium dioxide [J]. Journal of the European Ceramic Society, 2000, 2(1): 15-22.
[6] WOO Y C, KANG H J, KIM D J. Formation of TiC particle during carbothermal reduction of TiO2 [J]. Journal of the European Ceramic Society, 2007, 27(2/3): 719-722.
[7] KOC R, MENG C, SWIFT G A. Sintering properties of submicron TiC powders from carbon coated titania precursor [J]. Journal of Materials Science, 2000, 35(12): 3131-3141.
[8] PREISS H, BERGER L M, SCHULTZE D. Studies on carbothermal preparation of titanium carbide from different gel precurous [J]. Journal of the European Ceramic Society, 1999, 19(2): 195-206.
[9] ZHU Xin-kun, ZHAO Kun-yu, CHENG Bao-chang, LIN Qiu-shi, ZHANG Xiu-qing, CHEN Tie-li, SHU Yun-sheng, YONG QI-long. Fabrication of nanocrystalline TiC powder by high-energy ball milling [J]. The Chinese Journal of Nonferrous Metals, 2001, 11(2): 269-272. (in Chinese)
[10] MUNIR Z A. Synthesis of high temperature materials by self-propagating combustion methods [J]. American Ceramic Society Bulletin, 1988, 67 (2): 56-63.
[11] BAVBANDE D V, MISHRA R, JUNEJA J M. Studies on the kinetics of synthesis of TiC by calciothermic reduction of TiO2 in presence of carbon [J]. Journal of Thermal Analysis and Calorimetry, 2004, 78(3): 775-780.
[12] LEE D W, ALEXANDROVSKII S, KIM B K. Mg-thermal reduction of TiCl4+CxCl4 solution for producing ultrafine titanium carbide [J]. Materials Chemistry and Physics, 2004, 88(1): 23-26.
[13] WEIMER A W. Carbide, nitride and boride materials-synthesis and processing [M]. London: Chapman & Hall, 1997.
[14] AFIR A, ACHOUR M, SAOULA N. X-ray diffraction study of Ti-O-C system at high temperature and in a continuous vacuum [J]. Journal of Alloys and Compounds 1999, 288(1/2): 124-140.
[15] XU Bao-qiang, YANG Bin, HE Jian-ping, SEN Wei, DAI Yong-nian, LIU Da-chun. Study on carbothermic reduction of titanium dioxide in vacuum [C]//Ba De-chun. Proceeding of 9th Vacuum Metallurgy and Surface Engineering Conference. Beijing: Electronics Industry Press, 2009: 499-505.
[16] YE Da-lun, HU Jian-hua. Practical data handbook of inorganic thermodynamics [M]. 2nd edition. Beijing: Metallurgical Industry Press, 2002: 6-25, 48-62. (in Chinese)
[17] KOC R. Kinetics and phase evolution during carbothermal synthesis of titanium carbide from carbon-coated titania powder [J]. Journal of the European Ceramic Society, 1997, 17(11): 1309-1315.
[18] OUENSANGA A. Thermodynamic study of the Ti-C-O system in the temperature range 1 400-1 600 K [J]. Journal of Less-Common Metals, 1979, 63(2): 225-235.
[19] LYUBIMOV V D, ALYAMOVSKII S I, SHVEIKIN G P. Mechanism of the reduction of titanium oxides by carbon [J]. Russian Journal of Inorganic Chemistry, 1981, 26(9): 1243-1247.
[20] YANG Yu-xing, QI Rui. X-ray diffraction analysis [M]. Shanghai: Shanghai Jiao-tong University Press, 1994: 129. (in Chinese)
[21] LI Shu-tang. Base of crystallology about X-ray diffraction [M]. Beijing: Metallurgical Industry Press, 1990: 154-155. (in Chinese)
[22] RAZAVI M, RAHIMIPOUR M R, KABOLI R. Synthesis of TiC nanocomposite powder from impure TiO2 and carbon black by mechanically activated sintering [J]. Journal of Alloys and Compounds, 2008, 460(1/2): 694-698.
[23] LOHSE B H, CALKA A, WEXLER D. Effect of starting composition on the synthesis of nanocrystalline TiC during milling of titanium and carbon [J]. Journal of Alloys and Compounds, 2005, 394(1/2): 148-151.
[24] DAI Yong-nian. Binary alloy phase diagrams [M]. Beijing: Science Press, 2009: 254. (in Chinese)
真空碳热还原法制备碳化钛粉末
森 维1, 2, 3, 徐宝强1, 2, 杨 斌1, 2, 孙红燕1, 2 , 宋建勋1, 2, 万贺利 1, 2, 戴永年1, 2
1. 真空冶金国家工程实验室,昆明 650093;
2. 云南省有色金属真空冶金重点实验室,昆明 650093;
3. 昆明理工大学材料科学与工程学院,昆明 650093
摘 要:采用XRD、SEM、XRF以及激光粒度分析仪等分析手段,研究真空条件下碳热还原TiO2制备的碳化钛粉末。热力学计算和实验结果表明:真空条件下容易获得碳化钛,且随温度逐渐升高得到的产物顺序为:Magneli相(Ti4O7)、Ti3O5、Ti2O3、TiCxO1-x和TiC。当物料配比为1:3.2-1:6时,在1 550 °C保温4 h的条件下可获得单相TiC粉末;物料配比为1:4和1:5时,产物粉末为标准化学计量的TiC1.0粉末;物料配比为1:4时,得到的产物为单相低杂质超细碳化钛粉末(D50为3.04 μm)、SEM观察表明在产物块体表面存在分布均匀、团聚小、结构疏松的结构。
关键词:二氧化钛;碳化钛;真空;碳热还原方法
(Edited by LAI Hai-hui)
Foundation item: Project(u0837604) supported by the Natural Science Foundation of Yunnan Province, China; Project(51004058) supported by the National Natural Science Foundation of China; Project(20095314110003) supported by Specialized Research Fund for the Doctoral Program of Higher Education
Corresponding author: YANG Bin; Tel: +86-871-5161583; E-mail: kgyb2005@126.com
DOI: 10.1016/S1003-6326(11)60697-3