
Effect of activated carbon and electrolyte on properties of supercapacitor
ZHOU Shao-yun(周邵云), LI Xin-hai(李新海), WANG Zhi-xing(王志兴),
GUO Hua-jun(郭华军), PENG Wen-jie(彭文杰)
School of Metallurgical Science and Engineering, Central South University, Changsha 410083, China
Received 15 July 2007; accepted 10 September 2007
Abstract: Effect of activated carbon and electrolyte on electrochemical properties of organic supercapacitor was investigated. The results show that specific surface area and mesoporosity of activated carbon influence specific capacitance. If specific surface area is larger and mesoporosity is higher, the specific capacitance will become bigger. Specific surface area influences resistance of carbon electrode and consequently influences power property and pore size distribution. If specific surface area is smaller and mesoporosity is higher, the power property will become better. Ash influences leakage current and electrochemical cycling stability. If ash content is lower, the performance will become better. The properties of supercapacitor highly depend on the electrolyte. The compatibility of electrolyte and activated carbon is a determining factor of supercapacitor’s working voltage. LiPF6/(EC+EMC+DMC) is inappropriate for double layer capacitor. MeEt3NPF4/PC has higher specific capacitance than Et4NPF4/PC because methyl’s electronegativity value is lower than ethyl and MeEt3N+ has more positive charges and stronger polarizability than Et4N+ when an ethyl is substituted by methyl.
Key words: activated carbon; specific surface area; mesoporosity; ash; electrolyte; compatibility; supercapacitor
1 Introduction
Supercapacitor is a kind of new energy storage device between secondary battery and physical capacitor. As a new device with great application prospect, it has become a research hotspot in recent years for its friendliness to environment, high safety, good power property and cycling performance. At present, because of its stable electrochemical behavior, good cycling performance and low cost, activated carbon has become a mainstream electrode material for commercial supercapacitors. The storage of energy exists in the double layer at the electrode/electrolyte interface[1]. The characteristic of double layer influences the performance of the whole supercapacitor and it depends on specific surface area, pore size distribution, ash content of activated carbon[2], and certain qualities of electrolyte such as stability, decomposition voltage, concentration, ionic diameter, electronegativity and the compatibility with activated carbon[3]. At present, the electrolytes of organic supercapacitor are mostly quaternary ammonium salts of tetrafluoro boric acid or hexafluoro phosphoric acid, and lithium salts for hybrid supercapacitor. CHMIOLA et al[4] have investigated the effect of pore and surface area of carbide derived carbons on specific capacitance in H2SO4 aqueous system, and their conclusion highlights the fact that the pore size and the pore shape or tortuousity can influence the storage of energy, however, they have not researched on the organic system, in which stricter pore size distribution is required. LIU et al[5] have compared the performances of two electrolytes consisting of the same solute but different solvents including acetonitrile and propylene carbonate, and reached a conclusion that propylene carbonate based electrolyte is a promising alternative, but they have not made a further research on the electrolytes with different solutes and the same solvent.
The effect of activated carbon and electrolyte on electrochemical properties of organic supercapacitor was investigated in this study, in order to supply some references to supercapacitor’s material companies for producing materials and supercapacitor companies for choosing materials.
2 Experimental
2.1 Test on physical properties of activated carbon
Activated carbons were provided by Huaxian Company (China, marked as symbol “A”) and Kuraray Chemistry Company (Japan, marked as symbol “B”). Their physical properties are listed in Table 1. Specific surface area and pore size distribution were tested by a N2 Isothermal Adsorption Tester and calculated based on BET and BJH means. Laser granularity measurement was employed to detect the particle size. Ash content was tested according to Ref.[6].
Table 1 Physical properties of activated carbons
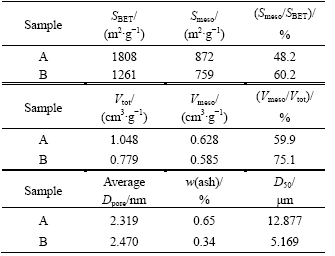
2.2 Preparation of electrode and assembly of coin- type capacitor
First, the activated carbon powders were mixed with 10% (mass fraction) carbon black and 5% PTFE in appropriate ethanol until slurry was obtained. Then, the blended slurries were pressed onto stainless steel current collector and cut to obtain electrode with diameter of 14 mm. Finally the electrode was dried at 120 ℃ for 6 h in vacuum. The test capacitor consists of two identical electrodes separated by a porous membrane, with 1 mol/L Et4NPF4/PC, MeEt3NPF4/PC, and LiPF6/(EC+ EMC+DMC) as electrolyte respectively. The assembly of the capacitors was carried out in a dry Ar-filled glove box.
2.3 Test on electrochemical performance of super- capacitor
AC impedance (10 kHz-1 mHz), cyclic voltam- metry (5 mV/s) and charge-discharge tests of super- capacitors were performed on a CHI660A Electro- chemical Workstation. Cycling performance was measured galvanostatically with a BS-9300 Secondary Batteries Tester (Qingtian, China).
3 Results and discussion
3.1 Pore structure analysis
Fig.1 shows N2 adsorption/desorption isotherms at 77.4 K of activated carbon A and B. Both of the activated carbons are found to give typical type-I nitrogen isotherms with an approximate smooth beeline at high relative pressure and a very sharp increase to reach a high adsorptive volume at low relative pressure. This indicates that they possess a lot of micropores. Adsorption/desorption isotherms of sample B have a clear hysteresis loop at high relative pressure, in contrast, sample A has no hysteresis, which shows sample B has more mesopores than sample A[7]. Besides, the same conclusion can also be drawn according to Smeso/SBET and Vmeso/Vtot in Table 1. Judged by adsorptive volume and cumulative pore volume, specific surface area of sample A is larger than that of sample B. The result indicates the high specific surface area of sample A is based on the precondition that it has more micropores.
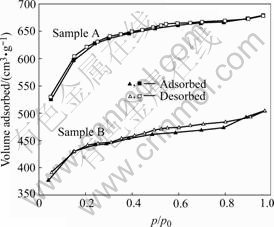
Fig.1 N2 adsorption/desorption isotherms of activated carbons
Fig.2 shows the pore size distribution curves of samples. For sample A, the pore size with maximal probability is 2.25 nm, and from 2 to 6 nm mesopores mainly distribute; and for sample B, besides the pores that are smaller than 1.95 nm, it has a focalization of pores at 3.75 nm. The average pore size of sample A is smaller than that of sample B. In Table 1, judged by Smeso/SBET and Vmeso/Vtot, the same result can also be achieved. Hence, sample B is more appropriate than sample A for the system with bigger electrolyte ions.
3.2 Effect of specific surface area and pore structure on capacitive behavior and power property
Cyclic voltammetry curves of both activated carbons are shown in Fig.3 (Electrolyte is Et4NPF4/PC). They do not show any major Faradic reactions within scan voltage window and have typical capacitive charac-
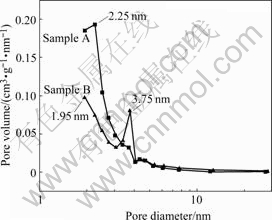
Fig.2 Pore size distribution of activated carbons
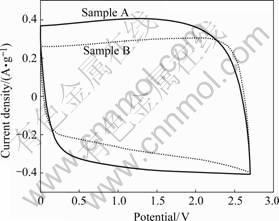
Fig.3 Cyclic voltammetry curves of activated carbons
teristics. According to the principle that specific capacitance keeps direct ratio with specific current at the same scan rate[8], sample A with higher specific surface area has larger specific capacitance gained from the plateau current of rectangle pattern; however, the same relationship between specific capacitance and BET specific surface area does not exist and increased specific surface area has not brought proportionate specific capacitance. This result ascribes that micropores with ultrafine pore size are difficult to be utilized by electrolyte ions and they are null surface area, so controlling pore size distribution in preparation process of activated carbon is quite important. At the moment the scan direction changes, if the current responds more quickly, the better capacitance and power properties of activated carbon can be gained. The hysteresis current of sample B is smaller than that of sample A, because electrolyte ions that filter in micropores are difficult to reverse quickly owing to small diffusion path and large surface tension[9].
Fig.4 shows frequency—specific capacitance curves calculated by AC impedance[10]. When AC signal frequency is high, specific capacitance is small because electrolyte ions can only form the double layer on the ektexine of activated carbon electrode. Specific capacitance increases gradually along with frequency lessening. Electrolyte ions can penetrate into the micropores; consequently, utility ratio of materials is improved. When AC signal frequency falls to a fixed value called Cut-off Frequency, surface area is utilized adequately and specific capacitance becomes invariable plateau. If the Cut-off Frequency is higher, the better power property and higher capacitance retention at large current density can be realized[11]. The relative large mesoporsity of sample B decides that its Cut-off Frequency is higher than that of sample A; as a result, its rate performance is better. Fig.5 shows specific capacitance at different current densities. When current density is low, sample A with higher specific surface area has larger specific capacitance, and it falls quickly because ions transfer velocity increases and micropores utility ratio declines as the current density increases. Relatively, specific capacitance of sample B falls mildly, and it is even higher than that of sample A at the high rate. Otherwise, the higher the specific surface area is, the more the pores will develop, accordingly, the resis-
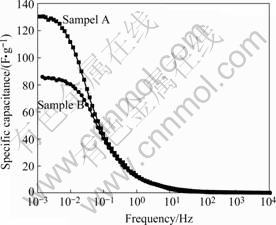
Fig.4 Frequency—specific capacitance curves of activated carbons
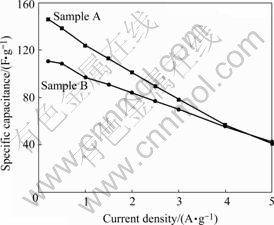
Fig.5 Relation between specific capacitance and current density
tance and polarization become bigger and the consumption of energy on resistance becomes greater. Thus it is essential to control specific surface area and mesoporosity of activated carbon.
3.3 Effect of ash on leakage current and cycling performance
Ash contents of samples A and B are 0.65% and 0.34%, respectively. Ash content is an important factor that influences the leakage current, self-discharge and cycling stability, and it can be reduced by means of strong acids process at the cost of reducing product’s yield and improving cost[12]. Fig.6 shows leakage current and self-discharge curves of activated carbons. Leakage current drops drastically before 15 min and stays at 1.5 mA and 0.75 mA, respectively. As a result of leakage current, open circuit voltage of supercapacitor reduces gradually with the extending of time, and it decreases quickly before 4 h and becomes gentle subsequently. The phenomenon can be explained by double layer consisting of compact layer that has strong interaction with electrode and diffusion layer that has weak interaction with electrode. This leads to biggish leakage current and self-discharge where diffusion layer ions regress to bulk solution at the beginning, and then compact layer ions to move to diffusion layer because of vibration and concentration difference. As the moving velocity is slow, leakage current and self-discharge keep relatively jarless and become small after some time. Ash can make these ions moving from double layer to bulk solution augment[13].
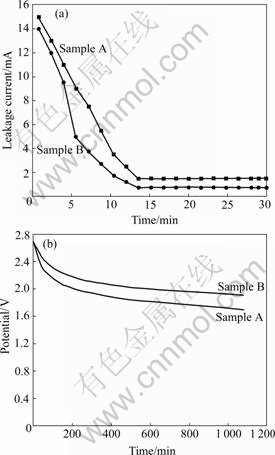
Fig.6 Leakage current (a) and self-discharge (b) curves of activated carbons
Ash can also influence cycling charge-discharge stability. Cycling performance experiments were conducted under condition of fluky room temperature, so there are some fluctuation of specific capacitance along with air temperature[14] from cycling performance curves in Fig.7. The capacitance fluctuation of sample A is larger and temperature stability is worse than that of sample B. There are also other influencing factors besides ash. For example, if there exist more mesopores, capacitance fluctuation with temperature will become smaller[15].
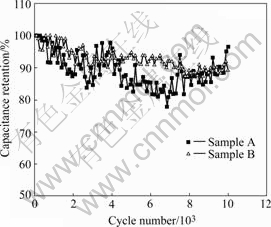
Fig.7 Cycling performance of activated carbons with different ash contents
3.4 Effect of electrolyte on capacitive behavior and power property
Activated carbon B with higher mesoporosity and better cycling stability is applied to determine the performance of three electrolytes, Et4NPF4/PC, MeEt3NPF4/PC and LiPF6/(EC+EMC+DMC). Cyclic voltammetry curves are shown in Fig.8. Et4NPF4/PC and MeEt3NPF4/PC possess wide potential windows with typical capacitive behavior; but potential window of LiPF6/(EC+EMC+DMC) is narrow, furthermore, its current response is slow when the scan direction changes, as a result, it influences the power property. In Fig.9, specific capacitance using LiPF6/(EC+EMC+DMC) as electrolyte drops fiercely while current densities are increased. Judged by this phenomenon and the later cycling performance, LiPF6/(EC+EMC+DMC) is claimed to be inappropriate for double layer capacitor. Because methyl’s electronegativity value is lower than ethyl and MeEt3N+ has more positive charges and stronger polarizability, which is more helpful for forming double layer than Et4N+ when an ethyl was substituted by methyl, plateau current of MeEt3NPF4/PC in Fig.8 and specific capacitance at different current densities in Fig.9 are bigger than those of Et4NPF4/PC[16].
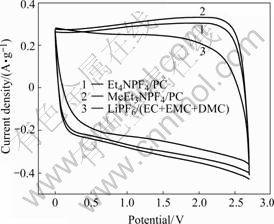
Fig.8 Cyclic voltammetry curves of activated carbon in different electrolytes
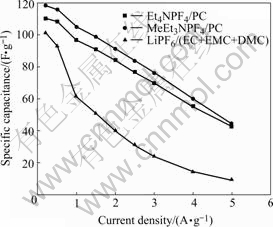
Fig.9 Relation between specific capacitance and current density in different electrolytes
3.5 Effect of compatibility of electrolyte and activated carbon on cycling performance
Besides electrolyte decomposition voltage, compatibility of electrolyte and activated carbon is an important factor influencing supercapacitor’s working voltage. For instance, decomposition voltage of LiPF6/ (EC+EMC+DMC) is higher than 4 V when it is used for lithium ion battery, but difficult to exceed 2.7 V used for double layer capacitor. Figs.10(a), (b) and (c) show the cycling performances charged at 2.7, 3.0 and 3.3 V, respectively. According to these figures, LiPF6/ (EC+EMC+DMC) decomposes at 2.7 V. At voltage higher than 3.0 V, supercapacitor explodes only a few charge-discharge cycle. Under 3.0 V, activated carbon and Et4NPF4/PC, MeEt3NPF4/PC can be compatible with each other well. Meanwhile, there are only a few electrolytes decomposed at 3.3 V, but no explosions happen after ten thousand cycles. It can also be found that cycling performance curves decline firstly and then rise when electrolytes decompose, for oxygen evolution can bring some capacity.
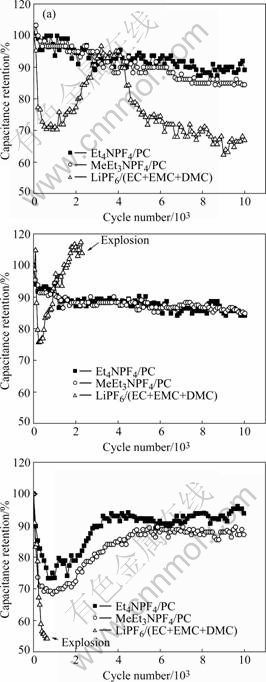
Fig.10 Cycling performance of activated carbon in different electrolytes: (a) 2.7 V; (b) 3.0 V; (c) 3.3 V
4 Conclusions
1) Specific surface area and mesoporosity of activated carbon influence specific capacitance. If specific surface area is larger and mesoporosity is higher, the specific capacitance will become bigger.
2) Specific surface area influences resistance of carbon electrode and consequently influences power property and pore size distribution. If specific surface area is larger and mesoporosity is higher, the power property will become better.
3) Ash influences leakage current and electrochemical cycling stability. If ash content is lower, the performance will become better.
4) The properties of supercapacitor highly depend on the electrolyte. The compatibility of electrolyte and activated carbon is a determining factor of working voltage of supercapacitor, LiPF6/(EC+EMC+DMC) is inappropriate for double layer capacitor. MeEt3NPF4/PC has higher specific capacitance than Et4NPF4/PC because electronegativity value of methyl is lower than that of ethyl, and MeEt3N+ has more positive charges and stronger polarizability than Et4N+ when an ethyl is substituted by methyl.
References
[1] ZHENG J P, JOW T R. A new charge storage mechanism for electrochemical capacitors [J]. J Electrochemical Society, 1995, 142(1): L6-L8.
[2] SHI F. Activated carbons and double layer capacitance [J]. Electrochimica Acta, 1996, 41(10):1633-1639.
[3] CONWAY B E. Electrochemical supercapacitors [M]. New York: Plenum Publishing, 1999: 155-164.
[4] CHMIOLA J, YUSHIN R, DASH R, GOGOTSI Y. Effect of pore size and surface area of carbide derived carbons on specific capacitance [J]. J Power Sources, 2006, 158(1): 765-772.
[5] LIU P, VERBRUGGE M, SOUKIAZIAN S. Influence of temperature and electrolyte on the performance of activated-carbon supercapacitors [J]. J Power Sources, 2006, 156(2): 712-718.
[6] BANSAL R C, DONNET J B, STOECKLI H F. Active carbon [M]. New York: Marcel Dekker, 1988: 95-96.
[7] GREGG S J, SING K S W. Adsorption, surface area and porosity [M]. London: Academic Press, 1982: 287.
[8] ?LVAREZ S, BLANCO L M C, MIRANDA O A J, FUERTES A B, CENTENO T A. Electrochemical capacitor performance of mesoporous carbons obtained by templating technique [J]. Carbon, 2005, 43(4): 866-870.
[9] SALITRA G, SOFFER A, ELIAD L, COHEN Y, AURBACH D. Carbon electrodes for double-layer capacitors (Ⅰ): Relations between ion and pore dimensions [J]. J Electrochem Soc, 2000, 147(7): 2486-2493.
[10] NIAN Y R, TENG H. Influence of surface oxides on the impedance behavior of carbon-based electrochemical capacitors [J]. J Electroanal Chem, 2003, 540(2): 119-127.
[11] K?TZ R, CARLEN M. Principles and applications of electrochemical capacitors [J]. Electrochimica Acta, 2000, 45: 2483-2498.
[12] NIAN Y R, TENG H. Nitric acid modification of activated carbon electrodes for improvement of electrochemical capacitance [J]. J Electrochem Soc, 2002, 149(8): A1008-A1014.
[13] TENG H, CHANG Y, HSIEH C. Performance of electric double-layer capacitors using carbons prepared from phenol-formaldeheyde resins by KOH etching [J]. Carbon, 2001, 39: 1981-1987.
[14] PANDOLFO A G, HOLLENKAMP A F. Carbon properties and their role in supercapacitors [J]. J Power Sources, 2006, 157(1): 11-27.
[15] RAFIK F, GUALOUS H, GALLAY R, CRAUSAZ A, BERTHON A. Frequency, thermal and voltage supercapacitor characterization and modeling [J]. J Power Sources, 2007, 165(2): 928-934.
[16] LI Hui. Coordination chemistry [M]. Beijing: Chemical Industry Press, 2006: 172-176.
(Edited by YANG Bing)
Foundation item: Project(2007CB613607) supported by the National Basic Research Program of China
Corresponding author: LI Xin-hai; Tel: +86-731-8836633; E-mail: zhoushaoyun2000@163.com