
ARTICLE
J. Cent. South Univ. (2019) 26: 1530-1539
DOI: https://doi.org/10.1007/s11771-019-4109-4

Lithium storage performance of {010}-faceted and [111]-faceted anatase TiO2 nanocrystals
DU De-jian(杜德健)1, DU Yi-en(杜意恩)1, 2, YUE Wen-bo(岳文博)1, YANG Xiao-jing(杨晓晶)1
1. Beijing Key Laboratory of Energy Conversion and Storage Materials, College of Chemistry,Beijing Normal University, Beijing 100875, China;
2. School of Chemistry & Chemical Engineering, Jinzhong University, Jinzhong 030619, China
Central South University Press and Springer-Verlag GmbH Germany, part of Springer Nature 2019
Abstract: As a popular anode material for lithium-ion batteries, anatase TiO2 nanoparticles with exposed {001} facets usually exhibit exceptional lithium storage performance owing to more accessible sites and fast migration of lithium ions along the good crystalline channels. However, there are few researches on the lithium storage capability of TiO2 nanocrystals with other high-energy facets owing to lack of effective synthesis method for controlling crystal facets. Herein, anatase TiO2 nanocrystals with exposed {010}- and [111]-facets are successfully prepared by using the delaminated tetratitanate nanoribbons as precursors. The electrochemical properties of these TiO2 nanocrystals with high-energy surfaces and the comparison with commercial TiO2 nanoparticles (P25) are studied. It is found that the cycle and rate performance of TiO2 nanocrystals is highly improved by reducing the particle size of nanocrystals. Moreover, TiO2 nanocrystals with exposed {010}- and [111]-facets exhibit better lithium storage capacities in comparison with P25 without a specific facet though P25 has smaller particle size than these TiO2 nanocrystals, indicating that the exposed facets of TiO2 nanocrystals have an important impact on their lithium storage capacity. Therefore, the synthesis design of high-performance TiO2 materials applied in the next-generation secondary batteries should both consider the particle size and the exposed facets of nanocrystals.
Key words: titanium dioxide; nanocrystal; exposed facet; lithium-ion battery
Cite this article as: DU De-jian, DU Yi-en, YUE Wen-bo, YANG Xiao-jing. Lithium storage performance of {010}-faceted and [111]-faceted anatase TiO2 nanocrystals [J]. Journal of Central South University, 2019, 26(6): 1530-1539. DOI: https://doi.org/10.1007/s11771-019-4109-4.
1 Introduction
As a popular n-type semiconductor electrode material, titanium dioxide (TiO2) has been potentially applied in solar cells, sensors, sodium- ion batteries and lithium-ion batteries (LIBs) owing to its low cost, robust capability, good safety and environmental friendliness [1-3]. Moreover, the volume change of TiO2 upon Li-ion intercalation and deintercalation is below 4%, which favors the safety and cycling stability of LIBs [4]. The insertion reaction of Li+ into TiO2 is expressed as follows: TiO2 + xLi+ + xe- → LixTiO2 (x≈0.5), and this reaction is kinetically controlled by the solid-state diffusion of Li+ [5]. Therefore, the crystal phase, particle size, morphology and surface structure of TiO2 can significantly affect its electrochemical performance. For instance, the small particle size and high specific surface area of TiO2 nanocrystals offer increased interfacial contact area with electrolyte and shorten the diffusion length for lithium ions, thereby highly enhancing the lithium storage capacity of TiO2 [6-8]. Moreover, some recent reports indicate that the exposed planes of TiO2 may also play an important part in lithium storage in nanostructured TiO2 [9-13]. To date, anatase TiO2 with exposed {001} facets has been widely studied due to its higher surface energy (0.90 J/m2) in comparison with {101} and {010} facets (0.44 and 0.53 J/m2) [14]. Compared to anatase TiO2 with exposed {101} facets, anatase TiO2 with exposed {001} facets exhibits superior lithium storage capability because of more accessible sites available and fast diffusion of lithium ions along the good crystalline channels (i.e., [001] direction) [9-13]. Moreover, a high Coulombic efficiency and remarkable capacity retention are also achieved for anatase TiO2 with exposed {001} facets. However, there are few researches on the electrochemical properties of TiO2 nanoparticles with other high-energy facets such as {010} facets so far [15].
Recently, Feng’s group and our group cooperatively reported a novel synthesis of {010}- faceted and [111]-faceted anatase nanoparticles by using exfoliated tetratitanate nanoribbons as precursors [16, 17]. The particle sizes and the exposed facets of TiO2 nanocrystals are easily controlled by changing pH values of solutions. Furthermore, it is interesting to find that the photocatalytic activities of these TiO2 catalysts increase in the order of surface without a specific facet (commercial P25)<[111]-faceted surface< {010}-faceted surface, indicating a serious effect of the exposed facets on the photocatalytic properties of anatase TiO2 [16]. It is reasonable that TiO2 nanocrystals with various exposed facets would also exhibit different electrochemical performance. In this work, {010}-faceted and [111]-faceted anatase TiO2 nanoparticles with various morphologies are prepared for studying the relationship between the exposed facets and the lithium storage property of TiO2 nanocrystals. The results suggest that the crystalline phase, particle size, morphology and exposed facets are highly depended on the pH values of solutions. As a result, nanorod-shaped anatase TiO2 nanocrystals with exposed [111]-facets are formed at pH 3, while rhombic-/cuboid-shaped and spindle-shaped anatase TiO2 nanocrystals with expose {010}-facets are obtained at pH 5 and 7, respectively. To conveniently represent these TiO2 nanocrystals, the samples are denoted as TiO2-T, where T is the pH values (3, 5 and 7). The electrochemical tests indicate that TiO2-3 exhibits better cycle and rate performance than TiO2-5 and TiO2-7 due to its small particle size. The large contact area between TiO2-3 and electrolyte is favorable to the diffusion and intercalation of lithium ions. However, although P25 has smaller particle size than TiO2-T, these anatase TiO2 nanocrystals with exposed {010}- and [111]-facets still exhibit better lithium storage capacity than P25 without a specific facet. It is inferred that lithium ions are more facile to be attached to the high-energy facets of TiO2 nanocrystals, resulting in the electrochemical performance improvement of TiO2-T. Consequently, the electrochemical property of anatase TiO2 can be remarkably increased by controlling not only the particle size and morphology, but also the exposed facets of TiO2.
2 Experimental
2.1 Sample preparation
2.1.1 Tetratitanate nanoribbon
Potassium tetratitanate fibers (K2Ti4O9) with layered structure were firstly immersed in 1 L of HCl solution (1 mol/L) with stirring for three days to obtain a protonated H2Ti4O9·nH2O (HTO) [16]. The acid exchange was repeated more than three times. The product was then collected by centrifugation and dried by vacuum freezing. The TMA+-intercalated tetratitanate compound (TMA-HTO) was prepared by immersing the HTO sample (5.7 g) in tetramethylammonium hydroxide (TMAOH) solution (57 mL, 12.5%) and then calcination at 100 °C for 24 h. The as-synthesized TMA-HTO was dispersed in 570 mL of distilled water with stirring for 24 h. A colloidal suspension (exfoliated HTO) was collected after removing the large particles.
2.1.2 Anatase with various facets
The pH value of the colloidal solution was tuned to 3, 5 and 7 respectively by adding a HCl or TMAOH solution. The solutions (60 mL) with different pH were then transferred into a 100 mL Teflon-lined autoclave and heated at 170 °C for 24 h. After the autoclave was cooled down, TiO2 nanocrystals were obtained after centrifugation and freeze-drying.
2.2 Sample characterization
Crystalline characterizations of samples were performed by X-ray diffraction (XRD) on a Phillips X’pert Pro MPD diffractometer. The morphologies and structures of samples were observed by scanning electron microscope (SEM) on a JEOL JSM-6700F with a voltage of 5 kV, transmission electron microscopy (TEM) and high-resolution TEM (HRTEM) on a JEOL JEM-2011 electron microscope with a voltage of 200 kV.
2.3 Electrochemical measurements
The electrochemical properties of samples were measured by using a LR2032-type coin cell. The negative electrode was Li metal and the positive electrode was a mixed slurry on a copper foil, including active materials (80%), super P carbon black (10%) and polyvinylidene difluoride (PVDF, 10%), and N-methyl-2-pyrrolidine (NMP) was used as solvent. 2.5 mg/cm2 mass loading is necessary for active materials. The electrolyte was prepared by dissolving LiPF6 (1 mol/L) in a mixed solution (EC:DEC:DMC with a 1:1:1 volume ratio). Coin cell was assembled in a glove box filled with Ar. Cyclic voltammetry and electrochemical impedance spectroscopy were tested on a Gamry Interface 1000 electrochemical station in the voltage range of 0.01-3.0 V (versus Li/Li+) with a scan speed of 0.5 mV/s or with a frequency range of 100 kHz to 10 mHz, respectively. LAND test system was used to measure the galvanostatic charge–discharge performance in the voltage range of 0.01-3.0 V under a constant current of 0.05–1 A/g.
3 Results and discussion
3.1 Materials characterization
Anatase TiO2 nanocrystals are prepared from as-synthesized HTO nanoribbons under hydrothermal treatment (see details in Section 2). According to our previous research, the crystalline phase (anatase single phase) is highly depended on the temperature (>150 °C) and pH (3-13) [16]. Herein, TiO2 nanocrystals are prepared at 170 °C in solutions with pH of 3, 5 and 7, respectively. The XRD patterns of these TiO2 nanocrystals are shown in Figure 1(a). All characteristic peaks in the XRD patterns are indexed into the tetragonal structure of anatase TiO2. Moreover, it is found that the morphology of the nanocrystals is also influenced by the pH values. The SEM image of TiO2-3 (Figure 1(b)) reveals that nanorod-like particles with an average size of about 60 nm in length and about 40 nm in width are formed at pH 3. At pH 5, two morphologies of TiO2-5, cuboid and rhombic shapes, can be found in the SEM image (Figure 1(c)). The size of mainly cuboid particles is about 70 nm in length and about 55 nm in width. The morphology of TiO2-7 changes to spindle shape when pH increases to 7 (Figure 1(d)), and the particle size increases to about 160 nm in length and about 55 nm in width. The particle size of these TiO2 nanocrystals was analyzed by using Nano Measurer software. The histogram of particle length based on statistical results (Figures 2(a)-(c)) shows that the average size of TiO2 nanocrystals is 58.8 nm for TiO2-3, 69.8 nm for TiO2-5 and 144.1 nm for TiO2-7. This means that the crystalline size increases with the increase of pH values under the same condition. Our previous study indicates that the cracks in the precursor [Ti4O9]2- nanoribbons appear along the [010]-direction of the HTO below pH 3 and along [001]-direction above pH 7, leading to the different morphologies and particle sizes of anatase TiO2 nanocrystals [16].
The morphologies and exposed facets of anatase TiO2 nanocrystals were further studied by TEM. TEM images also confirm the formation of TiO2 nanocrystals with a nanorod shape (Figure 3(a)), a cuboid or rhombic shape (Figure 3(c)) and a spindle shape (Figure 3(e)) respectively, which is in accord with the SEM observation. The nanocrystal in the HRTEM image of TiO2-3 (Figure 3(b)) exhibits lattice spacings of 0.352 and 0.352 nm, which are assigned to the (101) and (011) planes of TiO2 with an angle of ca. 82.1°. Therefore, the dominant exposed facets of TiO2-3 are perpendicular to [111] crystal zone axis, and denoted as [111]-facets. In contrast, the lattice spacings of 0.351 and 0.473 nm in the HRTEM image of TiO2-5 (Figure 3(d)) correspond to the (101) and (002) planes of TiO2 with an angle of ca. 68.3°. Therefore, the dominant exposed facets of TiO2-5 are the {010} facets and vertical to the [010] direction. The lattice spacings of 0.352 and 0.352 nm in the HRTEM image of TiO2-7(Figure 3(f)) correspond to the (101) and
planes of TiO2, and the angle between the two facets is 136.6°, also indicating that the {010} facets are the dominant exposed facets of TiO2-7. In a word, nanorod-shaped TiO2 nanoparticles with exposed [111]- facets were formed at pH 3, whereas rhombic-/cuboid- and spindle-shaped TiO2 nanoparticles with exposed {010}-facets were formed at pH 5, and 7, respectively.
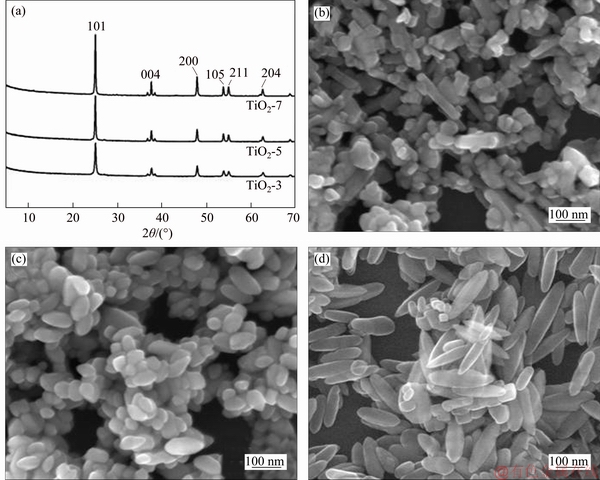
Figure 1 XRD patterns of TiO2-T (T=3, 5, 7) (a) and corresponding SEM images of TiO2-3 (b), TiO2-5 (c) and TiO2-7 (d)
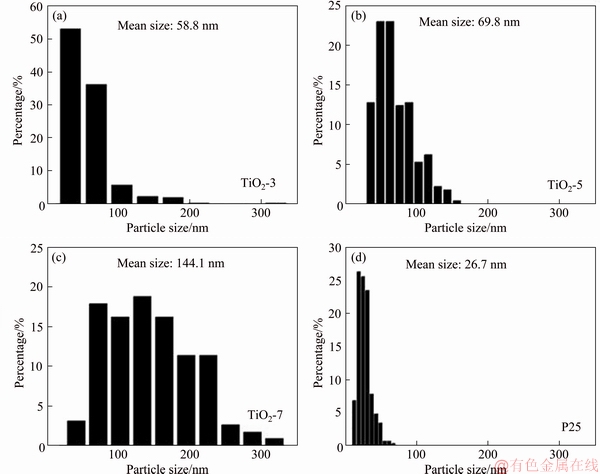
Figure 2 Particle size distribution of TiO2-3 (a), TiO2-5 (b), TiO2-7 (c) and P25 (d)
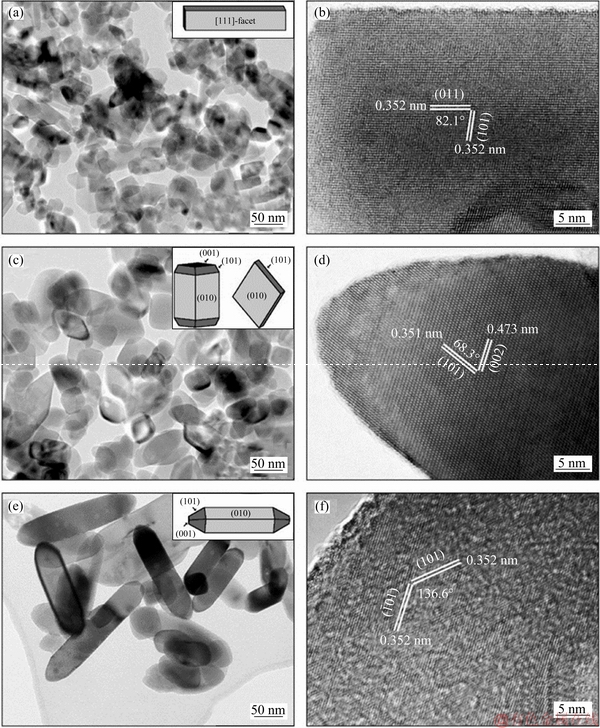
Figure 3 TEM and HRTEM images of TiO2-3 (a, b), TiO2-5 (c, d) and TiO2-7 (e, f) (Insets of (a, c, e) are structural models of TiO2-3, TiO2-5 and TiO2-7, respectively)
3.2 Electrochemical performance
The impact of the particle size and exposed facets on the electrochemical behaviors of anatase TiO2 nanocrystals was studied by cyclic voltammetry and galvanostatic measurements. Figures 4(a), (c), (e) show the cyclic voltammograms (CVs) of TiO2-3, TiO2-5 and TiO2-7 in the 1st, 2nd, 3rd and 6th cycles, respectively. There are two obvious cathodic and anodic peaks at about 1.5 and about 2.2 V, corresponding to the reversible transition from tetragonal anatase (I41/amd) to orthorhombic Li0.5TiO2 (Imma) [9, 12]. It is worth noting that the reduction and oxidation peaks still remain in the CV curves of TiO2-3 after the first cycle, whereas these peaks become inapparent in the CV curves of TiO2-5 and TiO2-7 with the increase of cycle number. The reduction peak at about 0.5 V is associated with the formation of solid electrolyte interface (SEI) films on the surface of TiO2 nanocrystals [18, 19]. This peak would disappear as the SEI films become stable. Figures 4(b), (d), (f) depicts voltage profiles of these TiO2 nanocrystals for the 1st, 2nd, 10th and 50th cycles. Two voltage plateaus at 1.7 and 2.1 V are observed in both charge and discharge curves of TiO2-3. On the contrary, the voltage plateaus are not distinct in the charge/discharge curves of TiO2-5 and TiO2-7, which is well-matched with the CV results. It is inferred that lithium ions are more facile to be attached to the high-energy facets of TiO2 nanocrystals, which is beneficial to the Li-ion intercalation and conversion reactions. In addition, according to the electrochemical properties of TiO2-5 and TiO2-7 with the same facets, the Li-ion intercalation performance is also influenced by the particle size, or in other words, the specific surface area of TiO2 nanocrystals.
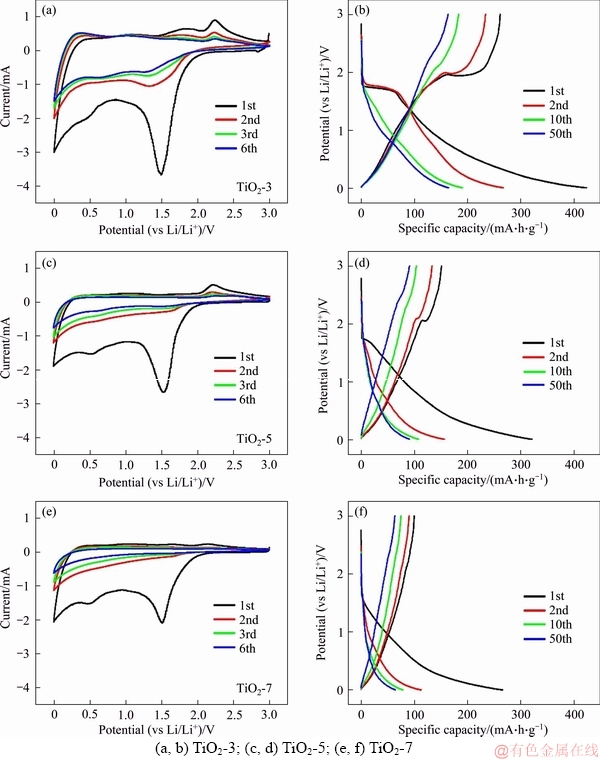
Figure 4 CV curves (a, c, e) and voltage profiles (b, d, f): (a, b) TiO2-3; (c, d) TiO2-5; (e, f) TiO2-7
The electrochemical properties of these TiO2 nanocrystals are also measured, and TiO2-3 shows the best cycle performance and rate capability among these TiO2 nanocrystals (Figures 5(a), (b)). For instance, the reversible capacity of TiO2-3 is maintained at about 160 mA h/g at 0.05 A/g over 100 cycles, which is comparable to the theoretical capacity of anatase TiO2 (167.5 mA·h/g). On the contrary, TiO2-5 and TiO2-7 deliver the capacities of only about 90 and about 60 mA·h/g respectively over 100 cycles. The discharge capacity of TiO2-3 is about 100 mA h/g as the current rate rises to 1 A/g, whereas the discharge capacities of TiO2-5 and TiO2-7 drop to about 60 and about 30 mA·h/g, respectively. The small particle size and large surface area of TiO2-3 can enhance the electrolyte/ electrode contact area and shorten lithium diffusion pathways, thereby improving the electrochemical performance of TiO2-3. It should be noted that the increased contact area also causes a larger SEI film formed on TiO2-3, which leads to more irreversible capacity losses and serious capacity fading in the initial cycles. The Coulombic efficiencies of TiO2-3, TiO2-5 and TiO2-7 are 59.3%, 39.8% and 41.5% in the first cycle respectively, and reach to above 98% after the 10th cycle. The low initial Coulombic efficiencies are attributed to the formation of SEI films on TiO2 nanocrystals and the irreversible generation of lithium titanate. It is interesting to note that TiO2-3 has an abnormal higher initial Coulombic efficiency than TiO2-5 and TiO2-7, implying that the growth behavior of SEI film is totally different on different faceted crystals [19]. Accordingly, in addition to the particle size, the exposed facet also plays a significant part in the electrochemical properties of TiO2 nanocrystals.
The electron and Li+ transport in these TiO2 nanocrystals were evaluated by using electrochemical impedance spectroscopy (EIS). Figure 5(c) shows the Nyquist plots consisting of a semicircle at the high frequency area, which is associated with the charge transfer resistance (Rct), and a sloping straight line at the low frequency area, which is related to the diffusion performance of Li+ ions in electrodes (Zw) [20, 21]. The Rct values of samples were obtained after fitting curves with the equivalent circuit model (Figure 5(d)). The charge transfer resistance of TiO2-3 is about 55.8 Ω, slightly lower than those of TiO2-5 (about 78.6 Ω) and TiO2-7 (about 87.1 Ω). The electron transfer relys on the magnitude of the band-gap, which is influenced by the particle size and defects of anatase [22]. More sub-band-gap states between the valence bands and conductions result in the lower charge-transfer resistance of TiO2-3. Therefore, TiO2-3 with lower resistance would display superior electrochemical performance as compared to other TiO2 samples [23].
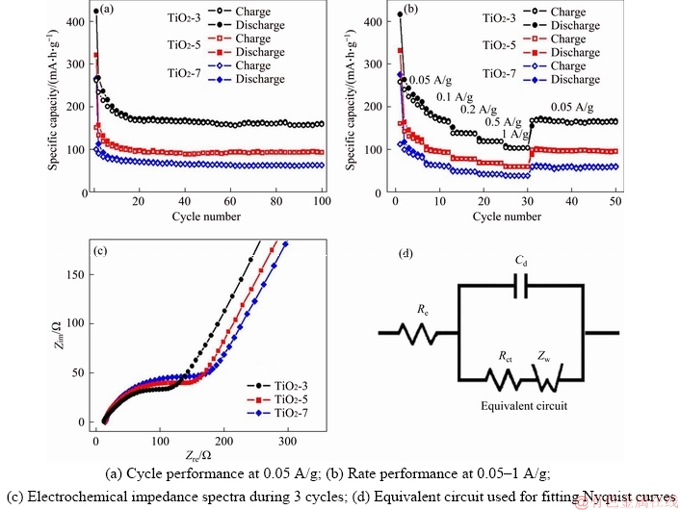
Figure 5 Electrochemical properties of TiO2-T:
3.3 Comparison with P25
Besides the particle size, the high-energy facets of TiO2 nanocrystals may also influence their electrochemical behavior. P25 is a well-known commercial TiO2 powder containing about 80% anatase phase and 20% rutile phase (Figure 6(a)) [24]. SEM and TEM images (Figures 6(b) and (c)) reveal that P25 are mostly irregular spherical nanocrystals without a specific facet on the surface. In a typical HRTEM image of P25 (Figure 6(d)), the lattice spacings of 0.351 and 0.241 nm are assigned to the (101) and (004) planes of TiO2 with an angle of ca. 68.3°, indicating the exposure of {010} facets on the basal plane. Furthermore, the (101) facets are parallel to the lateral faces of P25, indicating that the exposed facets are {101} on the lateral surface. The above results show that the small number of cuboid nanocrystals mainly expose {010} facets on the basal plane and {101} facets on the lateral surface. The main particle size of P25 is only 26.7 nm, much smaller than those of as-synthesized TiO2 nanocrystals with exposed {010}- and [111]-facets (Figure 2D).
In theory, P25 should exhibit superior electrochemical performance than these TiO2 nanocrystals due to its smaller particle size. However, the cycle and rate performance of P25 is merely comparable to those of TiO2-7 (Figure 7), implying that the exposed facet serves as an important factor for the lithium storage capacity of TiO2 as well. As reported previously, lithium ions prefer to be attached to the {010} facets of anatase rather than the {001} and {101} facets, and it is convenient for Li-ion transport along the [100] and [010] directions [25]. Therefore, TiO2-5 with exposed {010}-facets exhibit slightly better electrochemical performance than P25 though TiO2-5 has larger particle size. The [111]-facets are more complicated and their effects on the lithium transport need to be deeply studied in future.
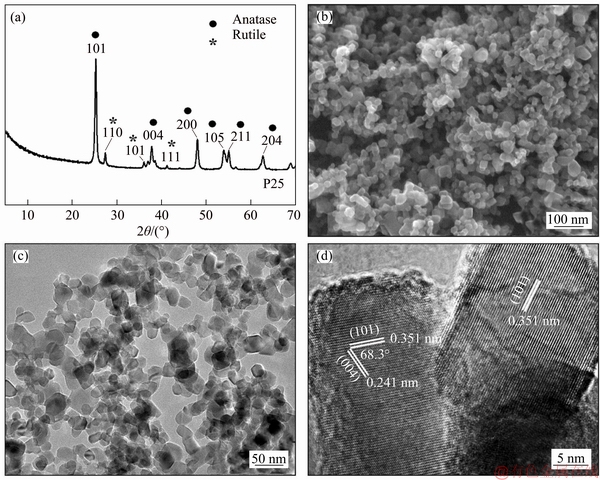
Figure 6 XRD pattern (a), SEM (b), TEM (c) and HRTEM images (d) of P25
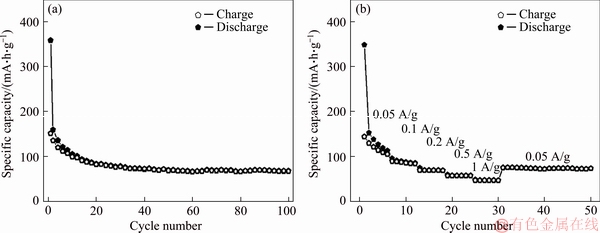
Figure 7 Cycle performance of P25 at 0.05 A/g (a) and rate performance of P25 at 0.05–1 A/g (b)
4 Conclusions
Anatase TiO2 nanocrystals with exposed {010}- and [111]-facets are successfully prepared by using exfoliated tetratitanate nanoribbons as precursors. The primary exposed facets of TiO2 nanoparticles are controllable by adjusting the pH values of reaction solution. The cycle and rate performance of these TiO2 nanocrystals are highly improved by reducing the particle size of nanocrystals. Moreover, these TiO2 nanocrystals exhibit better electrochemical performance than P25 without a specific facet though P25 has smaller particle size. These results reveal that the electrochemical properties of anatase TiO2 are affected not only by the crystal size and morphology, but also by the surface facets.
References
[1] SUBRAMANIAN V, KARKI A, GNANASEKAR K I, EDDY F P, RAMBABU B. Nanocrystalline TiO2 (anatase) for Li-ion batteries [J]. Journal of Power Sources, 2006, 159(1): 186-192. DOI: 10.1016/j.jpowsour.2006.04.027.
[2] HE Han-na, WANG Hai-yan, SUN Dan, SHAO Min-hua, HUANG Xiao-bing, TANG You-gen. N-doped rutile TiO2/C with significantly enhanced Na storage capacity for Na-ion batteries [J]. Electrochimica Acta, 2017, 236: 43-52. DOI: 10.1016/j.electacta.2017.03.104.
[3] HE Han-na, SUN Dan, ZHANG Qi, FANG Fu, TANG You-gen, GUO Jun, SHAO Min-hua, WANG Hai-yan. Iron doped cauliflower-like rutile TiO2 with superior sodium storage properties [J]. ACS Applied Materials & Interfaces, 2017, 9(7): 6093-6103. DOI: 10.1021/acsami.6b15516.
[4] DENG Da, KIM M G, LEE J Y, CHO J. Green energy storage materials: Nanostructured TiO2 and Sn-based anodes for lithium-ion batteries [J]. Energy & Environmental Science, 2009, 2(8): 818-837. DOI: 10.1039/B823474D.
[5] KAVAN L. Lithium insertion into TiO2 (anatase): electrochemistry, Raman spectroscopy, and isotope labeling [J]. Journal of Solid State Electrochemistry, 2014, 18(8): 2297-2306. DOI: 10.1007/s10008-014-2435-x.
[6] ARMSTRONG A R, ARMSTRONG G, CANALES J, BRUCE P G. TiO2–B nanowires as negative electrodes for rechargeable lithium batteries [J]. Journal of Power Sources, 2005, 146(1, 2): 501-506. DOI: 10.1016/j.jpowsour.2005. 03.057.
[7] ARMSTRONG G, ARMSTRONG A R, BRUCE P G, REALE P, SCROSATI B. TiO2(B) nanowires as an improved anode material for lithium-ion batteries containing LiFePO4 or LiNi0.5Mn1.5O4 cathodes and a polymer electrolyte [J]. Advanced Materials, 2006, 18(19): 2597-2600. DOI: 10.1002/adma.200601232.
[8] SHI Si-qi, GAO Jian, LIU Yue, ZHAO Yan, WU Qu, JU Wang-wei, OUYANG Chu-ying, XIAO Rui-juan. Multi-scale computation methods: Their applications in lithium-ion battery research and development [J]. Chinese Physics B, 2016, 25(1): 018212. DOI: 10.1088/1674- 1056/25/1/018212.
[9] CHEN Jun-song, TAN Yi-liang, LI Chang-ming, CHEAH Y L, LUAN De-yan, MADHAVI S, BOEY F Y C, ARCHER L A, LOU Xiong-wen, Constructing hierarchical spheres from large ultrathin anatase TiO2 nanosheets with nearly 100% exposed (001) facets for fast reversible lithium storage [J]. Journal of the American Chemical Society, 2010, 132(17): 6124-6130. DOI: 10.1021/ja100102y.
[10] SUN Cheng-hua, YANG Xiao-hua, CHEN Jun-song, LI Zhen, LOU Xiong-wen, LI Chun-zhong, SMITH S C, LU Gao-qing, Yang Hua-gui. Higher charge/discharge rates of lithium-ions across engineered TiO2 surfaces leads to enhanced battery performance [J]. Chemical Communications, 2010, 46(33): 6129-6131. DOI: 10.1039/C0CC00832J.
[11] YU Yan-long, WANG Xiao-liang, SUN Hong-yu, AHMAD M. 3D anatase TiO2 hollow microspheres assembled with high-energy {001} facets for lithium-ion batteries [J]. RSC Advances, 2012, 2(20): 7901-7905. DOI: 10.1039/ C2RA20718D.
[12] CHENG Xun-liang, HU Ming, HUANG R, JIANG Ji-sen, HF-free synthesis of anatase TiO2 nanosheets with largely exposed and clean {001} facets and their enhanced rate performance as anodes of lithium-ion battery [J]. ACS Applied Materials & Interfaces, 2014, 6(21): 19176-19183. DOI: 10.1021/am504971h.
[13] MING Hai, KUMAR P, YANG Wen-jing, FU Yu, MING Jun, KWAK W J, LI L J, SUN Y K, ZHENG Jun-wei, Green strategy to single crystalline anatase TiO2 nanosheets with dominant (001) facets and its lithiation study toward sustainable cobalt-free lithium ion full battery [J]. ACS Sustainable Chemistry & Engineering, 2015, 3(12): 3086-3095. DOI: 10.1021/acssuschemeng.5b00553.
[14] XU Hua, REUNCHAN P, OUYANG Shu-xin, TONG Hua, UMEZAWA N, KAKO T, YE Jin-hua. Anatase TiO2 single crystals exposed with high-reactive {111} facets toward efficient H2 evolution [J]. Chemistry of Materials, 2013, 25(3): 405-411. DOI: 10.1021/cm303502b.
[15] HAN Xi-guang, HAN Xiao, SUN Lin-qiang, WANG Po, JIN Ming-shang, WANG Xiao-jun. Facile preparation of hybrid anatase/rutile TiO2 nanorods with exposed (010) facets for lithium ion batteries [J]. Materials Chemistry and Physics, 2016, 171: 11-15. DOI: 10.1016/j.matchemphys.2015. 11.048.
[16] DU Yi-en, FENG Qi, CHEN Chang-dong, TANAKA Y, YANG Xiao-jing. Photocatalytic and dye-sensitized solar cell performances of {010}-faceted and [111]-faceted anatase TiO2 nanocrystals synthesized from tetratitanate nanoribbons [J]. ACS Applied Materials & Interfaces, 2014, 6(18): 16007-16019. DOI: 10.1021/am503914q.
[17] DU Yi-en, DU De-jian, FENG Qi, YANG Xiao-jing. Delithation, exfoliation, and transformation of rock-salt- structured Li2TiO3 to highly exposed {010}-faceted anatase [J]. ACS Applied Materials & Interfaces, 2015, 7(15): 7995-8004. DOI: 10.1021/acsami.5b00227.
[18] PFANZELT M, KUBIAK P, FLEISCHHAMMER M, WOHLFAHRT-MEHRENS M. TiO2 rutile–An alternative anode material for safe lithium-ion batteries [J]. Journal of Power Sources, 2011, 196(16): 6815–6821. DOI: 10.1016/ j.jpowsour.2010.09.109.
[19] ZHANG Qi, HE Han-na, HUANG Xiao-bing, YAN Jun, TANG You-gen, WANG Hai-yan. TiO2@C nanosheets with highly exposed (001) facets as a high-capacity anode for Na-ion batteries [J]. Chemical Engineering Journal, 2018, 332: 57-65. DOI: 10.1016/j.cej.2017.09.044.
[20] ZHAO Peng, YUE Wen-bo, YUAN Xu, BAO Hua-ying. Exceptional lithium anodic performance of Pd-doped graphene-based SnO2 nanocomposite [J]. Electrochimica Acta, 2017, 225: 322–329. DOI: 10.1016/j.electacta.2016. 12.124.
[21] ZHANG Si-qi, LIN Rong, YUE Wen-bo, NIU Fang-zhou, MA Jie, YANG Xiao-jing. Novel synthesis of metal sulfides-loaded porous carbon as anode materials for lithium-ion batteries [J]. Chemical Engineering Journal, 2017, 314: 19-26. DOI: 10.1016/j.cej.2016.12.123.
[22] ABBASI A, MIRHABIBI A, ARABI H, GOLMOHAMMAD M, BRYDSON R. Synthesis, characterization and electrochemical performances of γ-Fe2O3 cathode material for Li-ion batteries [J]. Journal of Materials Science: Materials in Electronics, 2016, 27(8): 7953-7961. DOI: 10.1007/s10854-016-4788-7.
[23] XUE Xia, SUN Dan, ZENG Xian-guang, HUANG Xiao-bing, ZHANG He-he, TANG You-gen, WANG Hai-yan. Two-step carbon modification of NaTi2(PO4)3 with improved sodium storage performance for Na-ion batteries [J]. Journal of Central South University, 2018, 25(10): 2320-2331. DOI: 10.1007/s11771-018-3916-3.
[24] CHEN Chang-dong, XU Lin-feng, SEWVANDI G A, KUSUNOSE T, TANAKA Y, NAKANISHI S, FENG Qi. Microwave-assisted topochemical conversion of layered titanate nanosheets to {010}-faceted anatase nanocrystals for high performance photocatalysts and dye-sensitized solar cells [J]. Crystal Growth & Design, 2014, 14(11): 5801- 5811. DOI: 10.1021/cg501062r.
[25] YANG Xu-ming, WANG Chao, YANG Ying-chang, ZHANG Yan, JIA Xin-nan, CHEN Jun, JI Xiao-bo. Anatase TiO2 nanocubes for fast and durable sodium ion battery anodes [J]. Journal of Materials Chemistry A, 2015, 3(16): 8800-8807. DOI: 10.1039/C5TA00614G.
(Edited by FANG Jing-hua)
中文导读
具有{010}和[111]晶面的锐钛矿型TiO2纳米晶体的储锂性能
摘要:作为锂离子电池的负极材料,具有{001}晶面的锐钛矿型TiO2纳米晶体通常展现出良好的储锂性能,这是由于该晶面具有更多的接触位点,同时锂离子沿着该晶格方向的扩散速度较快。然而,由于缺乏有效的控制TiO2晶面的合成方法,对具有其它高能晶面TiO2晶体的储锂性能的研究较少。本文以剥离的钛酸盐纳米带为前驱体,通过水热法合成了具有{010}和[111]晶面的锐钛矿型TiO2纳米晶体。通过对该TiO2纳米晶体的电化学性能进行研究,并与商业用的TiO2纳米颗粒(P25)的性能进行比较,发现通过减小TiO2纳米晶的粒径,可以大幅度提高TiO2纳米晶体的循环和倍率性能。此外,尽管P25具有更小的粒径,相比没有特定晶面的P25,具有{010}和[111]晶面的TiO2纳米晶体仍展现出更好的储锂能力。这表明TiO2纳米晶体的暴露晶面对其储锂性能同样具有重要影响。因此,在设计合成具有高性能的TiO2电极材料时,要同时考虑纳米晶体的粒径和暴露晶面等因素。
关键词:二氧化钛;纳米晶体;暴露晶面;锂离子电池
Foundation item: Projects(21573023, 51572031) supported by the National Natural Science Foundation of China; Project supported by the Grants-in-Acid for Doctor Research Funds; Project supported by “1331 Project” for Innovation Team Construction Plan Funds of Jinzhong University, China; Project supported by “1331 Project” for 2018 Key Innovation Team Construction Plan Funds of Shanxi Province, China
Received date: 2018-12-05; Accepted date: 2019-02-02
Corresponding authors: YUE Wen-bo, PhD, Professor; Tel: +86-10-58804229; E-mail: wbyue@bnu.edu.cn; ORCID: 0000-0003-3809- 6733; YANG Xiao-jing, PhD, Professor; Tel: +86-10-58805476; E-mail: yang.xiaojing@bnu.edu.cn; ORCID: 0000-0003-3620-3507