
Effect of graphite content on electrochemical performance of Sn-SnSb/graphite composite powders
HUANG Ke-long(黄可龙), ZHANG Ge(张 戈), LIU Su-qin(刘素琴)
School of Chemistry and Chemical Engineering, Central South University, Changsha 410083, China
Received 26 September 2006; accepted 13 November 2006
Abstract: Sn-SnSb alloy was synthesized by reducing a aqueous solution containing Sn(Ⅱ) and Sb(Ⅲ) salts with NaBH4 in the presence of sodium citrate. The product was characterized by X-ray diffractometry(XRD) and scanning electron microscopy(SEM). Sn-SnSb/graphite composite powders were prepared by mechanical milling and the mass fraction of graphite was increased from 20% to 50%. The effect of graphite content on the electrochemical performance of Sn-SnSb/graphite composite electrode was investigated. The results show the increase of graphite content is in favor of enhancing the first charge-discharge efficiency and improving the cycle performance, but the capacity of the composite electrode decreases with increasing content of graphite.
Key words: Sn-SnSb/graphite; composite electrode; cycle performance; Li-ion battery
1 Introduction
Lithium-ion batteries have been widely used in our daily life. The most popular anodes are based on intercalative carbonaceous materials[1]. Although these anode materials have excellent cycling stability, the reversible capacity is normally below 370 mA?h/g. As alternatives, lithium storage metals, such as Sn, Sb, Si and Al have been researched because of much larger capacity. Large-capacity lithium-ion batteries with alloy anodes, however, have not penetrated into commercial markets so far. The major problem is a large volume change that accompanies lithium insertion and extraction, which causes rapid mechanical disintegration of the metallic host and subsequent degradation of electrode performance[2-6]. It is especially serious under high lithium utilization. In order to minimize the mechanical stress and volume effect, multi-phase composite structures and small particle alloy systems have been proposed[7-9].
On the other hand, carbonaceous materials experience small volume expansion for lithium accommodation (9% graphite) and have soft and flexible characteristics. Lithium storage metals and alloys in combination with carbon can endure the volume change and reduce the mechanical stress within the electrode, and thereby prevent the disintegration[10]. However, most of alloy/carbon composites are still inferior to carbonaceous materials in the cycleability, especially if metallic host with large insertion capacity is used as a component.
The storage lithium capacity of Sn-SnSb multi-phase alloy exceeds 800 mA?h/g, but the cycle performance is poor[11-12]. In this study, Sn-SnSb alloy was synthesized by chemically reductive precipitation in aqueous solution and Sn-SnSb/graphite composite materials were prepared by ball milling. And the effect of graphite content on the electrochemical performance of Sn-SnSb/graphite composite electrode was investigated.
2 Experimental
Sn/SnSb powders were prepared by reductive co-precipitation of metal chlorides from aqueous solution with NaBH4. To produce Sn/SnSb multiphase alloy, solution A containing Na3C6H5O7·2H2O (as a complexant) and SnCl2 and SbCl3 with molar ratio of Sn2+ to Sb3+ being 3?1, and solution B containing NaOH and NaBH4 (as reducing agent) were used. The pH value of solution B was about 12. Solution B was added dropwise to solution A under strong magnetic stirring. The resultant suspension was separated by centrifuge and rinsed several times using deionized water, dilute HCl and actone. Finally, the precipitate was dried in vaccum at 50 ℃ for 12 h.
To produce Sn-SnSb/graphite composite materials, the mixtures of Sn-SnSb and pure graphite with different mass fractions of graphite (20% and 50%) were put into stainless steel vial (100 mL), respectively. The mass ratio of ball to powders was 15?1. The vial was evacuated and then purged with pure argon. The ball milling was carried out in a planetary ball milling machine (ND-2L) at a rotation rate of 300 r/min for 10 h.
X-ray diffraction of the precipitated product was carried on a RigaKu D/max2550VB+18 kW using monochromatised Cu Kα radiation at a scan speed of 4 (?)/min and scan range of 10?-90?. The particle surface morphology of the precipitated product was observed on a JSM-6360-LV scanning electron microscope. The electrochemical performance of product was tested on LAND (CT2001A) test system.
To measure electrochemical performance, electrodes were prepared by coating mixture of Sn-SnSb/graphite composite powders (90%) and PTFE binder (10%) onto a 1 cm2 non-corrosive steel current collector. The electrodes were dried in a vacuum oven at 120 ℃ for 12 h then cold pressed at 20 MPa. The half-cells were assembled using argon-filled MBRAUN glove box, and the volume fractions of H2O and O2 were less than 10-6. The Sn-SnSb/graphite composite powders were used as the working electrode and lithium foils were used as the counter and reference electrodes. 1 mol/L LiPF6 and EC+DMC+EMC (volume ratio of 1?1?1) were used as the electrolyte. Electrodes were cycled between 10 and 1 500 mV (vs Li) at a constant current density of 0.4 mA/cm2.
3 Results and discussion
3.1 Physical characterization of Sn-SnSb/graphite composite powders
The XRD pattern of Sn-SnSb/graphite composite powder is shown in Fig.1. In contrast with JCPDS cards, the primary peaks indicate the presence of graphite (space group P63/mmc), rhombohedral β-SnSb (space group R-3m) phase and ductile Sn phase.
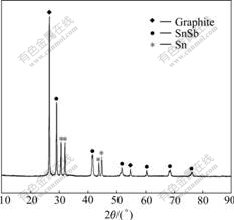
Fig.1 XRD pattern of Sn-SnSb/graphite composite powders
The SEM images of pure Sn-SnSb and Sn-SnSb/ graphite composite powders are shown in Fig.2. The prepared Sn-SnSb multi-phase alloy powders show some degree of agglomeration (see Fig.2(a)). The individual particle size of Sn-SnSb powder is estimated to be about 200 nm. Fig.2(b) shows that the black graphite uniformly distributes in Sn-SnSb powder. Fig.2(c) shows that the spherical graphite is surrounded by Sn-SnSb particles.
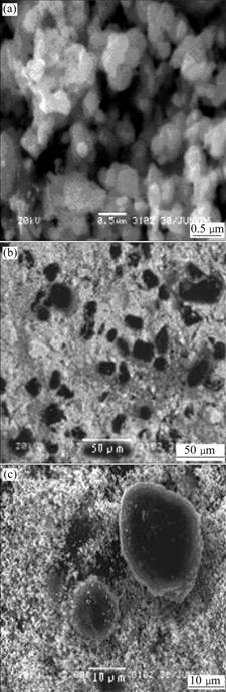
Fig.2 SEM images of prepared powders: (a) Pure Sn-SnSb; (b) and (c) Sn-SnSb/graphite composite powders ((c) is magnified image of (b))
3.2 Charge/discharge test of Sn-SnSb/graphite com- posite electrode
The first charge—discharge curves of graphite are shown in Fig.3. During the first discharge, the potential drops quickly from 1.90 to 0.25 V. A small plateau can be identified at about 0.8 V mainly due to the decom- position of electrolyte on electrode surface with the formation of SEI film[13]. The curve is flat in the range of 0.25-0.005 V, most of capacities are obtained within this voltage range as well as the first charge curve. The reversible capacity is obtained in the range of 0.25-0.005 V. The initial reversible capacity of graphite electrode is 341.1 mA·h/g.
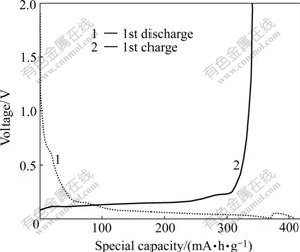
Fig.3 First charge—discharge curves of graphite at current density of 0.1 mA/cm2
The charge—discharge plots of Sn-SnSb/graphite with mass fractions of graphite of 20% are shown in Figs.4 and 5, respectively. ERLICH et al[14] reported that the Sn/SnSb electrode first reacted at about 0.8 V during Li insertion according to the reaction:
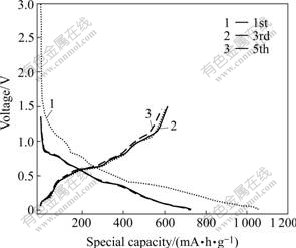
Fig.4 Charge—discharge curves of Sn-SnSb/graphite (20%) for 1st, 3rd and 5th cycles (current density: 0.25 mA/cm2)
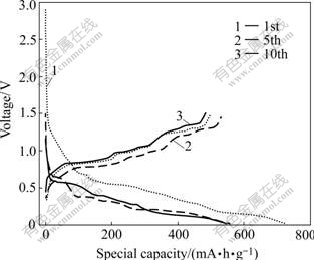
Fig.5 Charge—discharge curves of Sn-SnSb/graphite (50%) for 1st, 5th and 10th cycles (current density: 0.25 mA/cm)
SnSb+3Li++3e-
Li3Sb+Sn
WACHTLER et al[15] and REN et al[16]found that Li3Sb was first formed from SnSb alloy along with the segregation of Sn. When the potential was reduced below 0.7 V, Sn became active towards lithium, yielding various Li-Sn alloys of composition of LixSny. Within this voltage range, potential dropped slowly and no clearly defined plateaus could be identified for the electrode tested. This was mainly due to the multistep reactions of Li-Sn alloys. To Sn-SnSb/graphite composite electrode, graphite can also storage lithium in the range of 0.25-0.005 V. The initial charge and discharge specific capacities of Sn-SnSb/ graphite (20%) composite electrode are 1 00 8 and 605 mA·h/g, respectively (irreversible capacity: 403 mA?h/g). The first charge and discharge specific capacities of Sn-SnSb/graphite (50%) composite electrode are 731 and 501 mA·h/g, respectively. Therefore, it can be found that the capacity of the composite electrode reduces with increasing content of graphite. The electrode efficiencies of Sn-SnSb/graphite (20% and 50%) are shown in Fig.6. The first charge—discharge efficiencies of the two composite electrodes with mass fractions of graphite of 20% and 50% are 60.01% and 68.53%, respectively. This indicates that the increase of graphite content is in favor of enhancing the first charge—discharge efficiency. The reason for the large first irreversible capacity and low initial charge—discharge efficiency are as follows. Firstly, the reduction reaction of electrolyte takes place on electrode surface with the formation of SEI film. Secondly, the oxide layer of alloy surface reacts irreversibly with Li, resulting in the loss of Li. Thirdly, the electrode undergoes severe volume expansion during charge and discharge process, accordingly leading to the loss of electric contact between part of active materials, so Li in this part of active materials will be unable to be removed during the next de-intercalation reaction, namely “dead lithium”. Lastly, from the aspects of thermodynamics and kinetics, parts of Li are fixed in some interstice positions and unable to be removed during the intercalation reaction, or the diffusion rate of lithium in host materials is slow, thus resulting in the loss of Li.
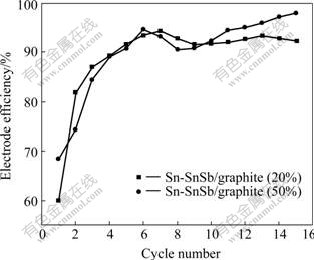
Fig.6 Electrode efficiencies of Sn-SnSb/graphite composite
3.3 Cycle performance of Sn-SnSb/graphite com- posite electrode
The cycle performances of Sn-SnSb/graphite (20% and 50%), Sn-SnSb and graphite are shown in Fig.7. The discharge capacity of Sn-SnSb/graphite (20%) composite electrode reaches the maximum of 615 mA·h/g in the 3rd cycle and that of Sn-SnSb/graphite (50%) reaches the maximum 536 mA·h/g in the 5th cycle. The discharge capacity of pure Sn-SnSb reaches the maximum of 653 mA·h/g in the 2nd cycle. This may be because the active electrode materials and the complete wetness of electrolyte need some time. After 15 cycles, the discharge specific capacities of Sn-SnSb/graphite (20% and 50%), pure Sn-SnSb and graphite are 461.0, 443.0, 337.0 and 333.6 mA·h/g, respectively. The fading rates of Sn-SnSb/graphite (20% and 50%), pure Sn-SnSb and graphite are 25%, 17%, 48% and 2.19%, respectively. The cycle performance of Sn-SnSb/graphite (50%) is better than that of Sn-SnSb/graphite (20%) regardless of the reversible capacity. The cycle performances of Sn-SnSb/graphite (20% and 50%) are superior to those of pure Sn-SnSb, mainly because graphite can effectively restrain the volume expansion of alloy during the lithium insertion and extraction. In addition, compared with graphite electrode, the join of Sn-SnSb multi-phase alloy greatly enhances the capacity of composite electrode, but the cycle performance is still inferior to graphite electrode.
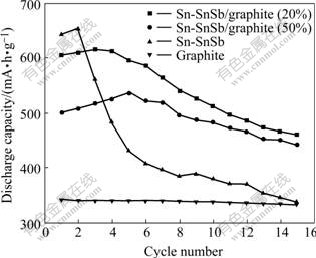
Fig.7 Cycle performances of Sn-SnSb/graphite (20% and 50%), pure Sn-SnSb and graphite
4 Conclusions
1) Sn-SnSb alloy powders are prepared by reductive co-precipitation using NaBH4 as reductant in the presence of sodium citrate. Sn-SnSb/graphite composite powders are prepared by ball milling and the mass fractions of graphite content are 20% and 50%, respectively.
2) The first charge—discharge efficiencies of the two composite electrodes with mass fractions of graphite of 20% and 50% are 60.01% and 68.53%, respectively. But the reversible capacity of the Sn-SnSb/graphite (20%) is higher than that of composite powder with mass fractions of graphite of 50%. After 15 cycles, the fading rates of Sn-SnSb/graphite (20% and 50%) are 25.00% and 17.00%, respectively. Sn-SnSb/graphite (50%) has a better cycle stability.
References
[1] TARASCON J M, ARMAND M. Issues and challenges facing rechargeable lithium batteries [J]. Nature, 2001, 414: 359-367.
[2] BESENHARD J O, YANG J, WINTER M. Will advanced lithium-alloy anodes have a chance in lithium-ion batteries? [J]. J Power Sources, 1997, 68: 87-92.
[3] FAUTEUX D, KOKSBANG R. Rechargeable lithium battery anodes: Alternatives to metallic lithium [J]. J Appl Electrochem, 1993, 23: 1-10.
[4] XIE J, ZHAO X B, CAO G S, ZHAO M J, SU S F. Electrochemical Li-uptake properties of nanosized NiSb2 prepared by solvothermal route [J]. Journal of Alloys and Compounds, 2005, 393: 283-286. (in Chinese)
[5] NEEDHAM S A, WANG G X, LIU H K. Electrochemical performance of SnSb and Sn/SnSb nanosize powders as anode materials in Li-ion cells [J]. Journal of Alloys and Compounds, 2005, 400: 234-238.
[6] HITOHIKO H, HIROKI S, ICHIRO T, TAKAO E. Anode behaviors of magnesium-antimony intermetallic compound for lithium secondary battery [J]. J Power Sources, 2003, 123: 216-221.
[7] YANG J, WACHTLER M, WINTER M, BESENHARD J O. Sub-microcrystalline Sn and Sn-SnSb powders as lithium storage materials for lithium-ion batteries [J]. Electrochem Solid-State Lett, 1999, 2: 161-163.
[8] KEPLER K D, VAUGHEY J T, THACKERAY M M. LixCu6Sn5 (0<x<13): An intermetallic insertion electrode for rechargeable lithium batteries [J]. Electrochem Solid-State Lett, 1999, 2: 307-310.
[9] ROM I, WACHTLER M, PAPAST I, SCHMIED M, BESENHARD J O, HOFER F, WINTER M. Electron microscopical characterization of Sn/SnSb composite electrodes for lithium-ion batteries [J]. Solid State Ionics, 2001, 143: 329-336.
[10] LI Hong, WANG Qing, SHI Li-hong. Nanosized SnSb alloy pinning on hard non-graphitic carbon spherules as anode materials for Li ion battery [J]. Chem Mater, 2002, 14: 103-108. (in Chinese)
[11] MUKAIBO H, OSAKA T, REALE P, PANERO S, SCROSATI B, WACHTLER M. Optimized Sn/SnSb lithium storage materials [J]. J Power Sources, 2004, 132(1/2): 225-228.
[12] TRIFONOVA A, WACHTLER M, WAGNER M R, SCHROETTNER H, HOFER F, WINTER M. Influence of the reductive preparation conditions on the morphology and on the electrochemical performance of Sn/SnSb [J]. Solid State Ionics, 2004, 168(1/2): 51-59.
[13] WU Y P, JIANG C, WAN C, HOLZE R. Mildly modified natural graphite as anode materials for lithium ion batteries [J]. J Power Sources, 2002, 111: 329-334. (in Chinese)
[14] ERLICH G M, DURAND C, CHEN X, HUGENER T A, SPIESS F, SUIB S L. Metallic negative electrode materials for rechargeable nonaqueous batteries [J]. J Electrochem Soc, 2000, 147: 886-891.
[15] WACHTLER M, WINTER M, BESENHARD J O. Anodic materials for rechargeable Li-batteries [J]. J Power Sources, 2002, 105: 151-160.
[16] REN Jian-guo, WANG Kan, HE Xin-ming, JIANG Chan-yin, WANG Cao-ren. Studies of alloy based anode materials for lithium ion batteries [J]. Progress in Chemistry, 2005, 17: 597-603. (in Chinese)
Foundation item: Project(50542004) supported by the National Natural Science Foundation of China; Project(20030533001) supported by the PFDP of the Education Ministry of China
Corresponding author: LIU Su-qin; Tel: +86-731-8879850; E-mail: zzg004@sina.com
(Edited by CHEN Wei-ping)