
Charge-discharge process of MnO2 supercapacitor
LIU Kai-yu(刘开宇)1, 2, ZHANG Ying(张 莹)1, ZHANG Wei(张 伟)1,
ZHENG He(郑 禾)1, SU Geng(苏 耿)1
1. School of Chemistry and Chemical Engineering, Central South University, Changsha 410083, China;
2. State Key Laboratory of Powder Metallurgy, Central South University, Changsha 410083, China
Received 15 September 2006; accepted 24 November 2006
Abstract: Mechanochemical synthesis of α-MnO2 was carried out with KMnO4 and Mn(CH3COO)2 in 1?1 mole ratio. The electrochemical performance of MnO2 electrode was investigated by cyclic voltammograms and alternating current impedance. The charge-discharge process of MnO2 supercapacitor in 6 mol/L KOH was studied within 1.2 V at 200 mA/g, suggesting that it displays double-layer capacibility in low potential scope and pseudo-capacitance properties in high potential scope. It is found that Mn3O4, an electrochemical inert, mainly forms in the initial 40 charge-discharge cycles. During cycling, the pseudo-capacitance properties disappear and the discharge curves are close to ideal ones, indicating double-layer capability. The maximum capacitance of MnO2 electrode is as high as 416 F/g, and retains 240 F/g after 200 cycles. The equivalent series resistance increases from 17 to 41 Ω.
Key words: manganese dioxide; supercapacitor; pseudo-capacitance; double-layer capability; charge-discharge process
1 Introduction
Compared with traditional capacitor, supercapacitor has higher capacitance, more energy density and power density, which makes it widely used in many kinds of fields from mobile equipment to electric vehicles[1]. Noble metal oxides such as RuO2 have high pseudocapacitance, but the application is limited by its high cost. However, some cheap metal oxides, such as Co3O4, NiO and MnO2, also have pseudocapacitance [2-3], and MnO2 has become hotspot in recent research for its abundant resource and good electrochemistry performance[4-5]. Studies on the preparation of MnO2 are always carried out with hydrothermal method[6], sol-gel method[7], circumfluence cooling method[8], etc. Nevertheless, the reports about mechanochemical synthesis of MnO2 are really few.
In this study, MnO2 was prepared mechano- chemically with KMnO4 and Mn(CH3COO)2 in 1?1 mole ratio, and level-loaded to a supercapacitor. The structure of as-prepared MnO2 was investigated by XRD and IR. Galvanostatic tests, cyclic voltammograms, and alternating current(AC) impedance were used, aiming at researching the performance of supercapacitor during charge-discharge cycling in 6 mol/L KOH.
2 Experimental
2.1 Reagents and instrument
Main reagents of KMnO4 (AR, Xiangke, Changsha), Mn(CH3COO)2·4H2O (AR, Xilong, Shantou), polytetra- fluoroethylene(PTFE) (5%, mass fraction) and acetylene black (Chelong, Shanxi) were used.
Main instruments of ND6-2L planetary ball-miller (Nadatianzun, Nanjing), D-500 X-ray diffractrometer (Siemens, Germany), CHI660 electrochemistry workstation (Chenhua, Shanghai)and Land battery tester (Jinnuo, Wuhan) were employed.
2.2 Experimental procedure
KMnO4 and Mn(CH3COO)2 were mixed in a stainless steel vessel in 1?1 mole ratio. After milling at 260 r/min for 9 h (the mass ratio of ball to power was 10?1), the product was pickled in 0.1 mol/L H2SO4, then stirred by magnetic force at 200 r/min for 2 h to remove K+. Subsequently, it was washed until neutral with distilled water to remove the redundant Mn(CH3COO)2. Then, it was dried at 120 ℃ to constant mass, mixed with acetylene black and PTFE (5%, mass fraction) at the mass ratio of 75?15?10, dried to subarid, rolled to electrode with 0.3 mm in depth, 10 mm×10 mm in area (stainless steel current collector). At last, it was dried at 120 ℃ and level-loaded to a symmetrical super- capacitor (Fig.1), using 6 mol/L KOH as the electrolyte.
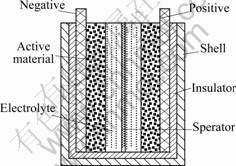
Fig.1 Schematic diagram of supercapacitor
The structure of as-prepared MnO2 was investigated using a Siemens D-500 X-ray diffractometer (Cu Kα radiation, 0.154 18 nm) in a θ/2θ geometry (XRD). Qualitative analysis of Mn—O and other functional groups were carried out with a FTIR (AVATAR360, Nicolet Corp, USA). Galvanostatic tests were performed on a Land CT2001A battery tester at 200 mA/g within 1.2 V, and manipulated by LANDdt 4.2 software. The cyclic voltammetry (CV, -0.60-0.65 V) and AC impedance (0.001 Hz-10 kHz) were operated in a three-electrode cell configuration. A Hg/HgO and a 2 cm×2 cm platinum gauze were used as reference and counter electrodes, respectively. In these studies, all electrodes were tested in 6 mol/L KOH solution.
3 Results and discussion
3.1 Structure characterization
The X-ray diffraction patterns of the MnO2 electrodes are shown in Fig.2. As can be seen from Fig.2 that the characteristic peaks of electrode material before charging appear at 12.78?, 36.94?, 37.52?, 41.22?, 41.9?, 49.8? and 69.7?, involving no peaks of other valent state manganese. All the peaks for the sample can be indexed to a pure tetragonal phase (space group I4/m (No.87)) of α-MnO2 (JCPDS 44-0141). The broaden terrace and low intension peaks indicate the MnO2 prepared is weak- crystalline[9-10]. After 200 charge-discharge cycles, the characteristic peaks at 32.41?, 36.04?, 44.37?, 58.76? as well as 37.52?, 41.9?, illuminate that MnO2 is turned into Mn3O4, leading to irreversible deep discharge.
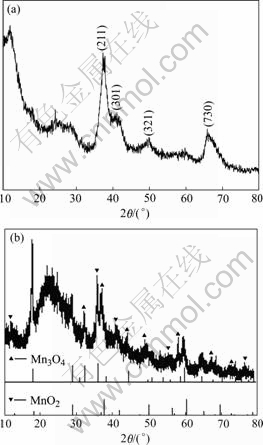
Fig.2 X-ray diffraction patterns of MnO2: (a) Before charge- discharge; (b) After 200 charge-discharge cycles
3.2 IR characterization
Fig.3 shows the IR pattern of the material. Peaks at about 3 374 cm-1 and 1 637 cm-1 correspond to the stretching and bending vibration of hydroxide in adsorbed water, and the ones at 1 383 cm-1 and 522 cm-1 indicate Mn—O bond characteristically[11]. Additionally, no other functional groups are involved.
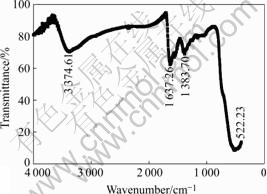
Fig.3 IR pattern of synthesized MnO2
3.3 Cyclic voltammograms and AC impedance
Typical cyclic voltammograms and AC impedance plots are shown in Figs.4 and 5, respectively.
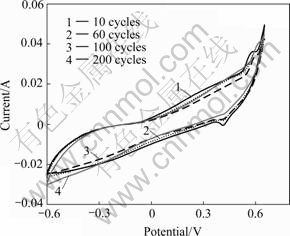
Fig.4 CV curves of MnO2 electrode at scan rate of 40 mV/s
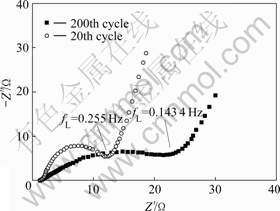
Fig.5 Nyquist plots for MnO2 electrode
At the beginning of scanning, a tiny oxidation peak appears at 0.57 V, and the corresponding reduction peak comes up at 0.41 V. With increasing number of scanning times, the redox peaks move positively. After 200 times of scanning, the oxidation peak moves to 0.62 V and the reduction one moves to 0.42 V, respectively. The dispersion is relevantly increased from 0.16 to 0.20 V, showing the irreversibility of electrode reaction. Meanwhile, the redox peaks disappear gradually, and the peak current diminishes gently. Mn3O4, an electro- chemistry inert, is the main cause of such phenomenon.
As shown in Fig.5, after 20 and 200 cycles, the AC impedance plots exhibit semi-circularities in the range of 0.001-10 kHz, indicating that the electrode processes are controlled by electrochemistry polarization, namely the proton embedding and disembodying interfacially. When the cycling numbers increase from 20 to 200, the semicircle diameter increases from 12 to 30 Ω, showing that the electrode reaction resistance increases. Besides, the exchange current can be calculated to be 430 mA/g and 172 mA/g respectively according to Ref.[12]. This is mainly caused by the production of Mn3O4 electro- chemistry inert material that reduces the surface catalytic activity of electrode.
Below 0.1 Hz, the biases are not ideally per- pendicular to the coordinates, indicating that the diffusion approach determines the electrode process, and the concentration dispersion polarization leads to the impedance. The slope may reflect the electric double-layer forming speed: the precipitous slope denotes fast electric double-layer forming speed. Fig.5 indicates that along with the increase of the cycle numbers, the formation speed of electric double-layer gets slower and slower, leading to the decline of electrode specific capacity.
3.4 Galvanostatic testing
Galvanostatic tests were carried out in 1.2 V potential window at 200 mA/g. Discharge curves after 2, 80 and 200 cycles are shown in Fig.6. Owning to the existence of equivalent series resistance(RESR), the voltage decreases suddenly at the beginning of discharging (Fig.6). RESR consists of the electrode/ solution, active material/current collector interface resistance and the electrochemistry reaction resistance.
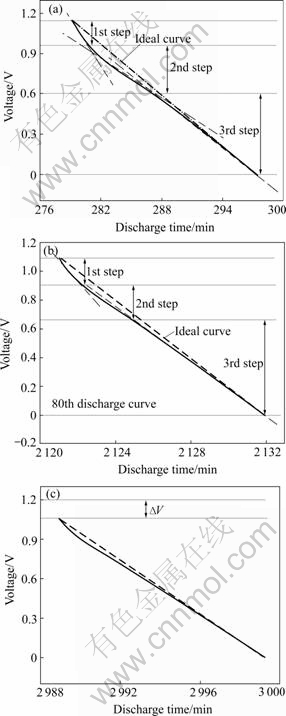
Fig.6 Discharge curves of MnO2 supercapacitor: (a) After 2 cycles; (b) After 80 cycles, (c) After 200 cycles
Supercapacitor shows different discharge characteristics in various voltage ranges. For example, the second discharge process experiences three steps (Fig.6(a)). The special linearity characteristic of electrochemistry double-layer capacitor displays in the low potential range of 0-0.6 V (the third step), as well as in high potential range of 0.95-1.15 V (the first step); but in the high potential range of 0.60-0.95 V (the second step), the flexural curve reflects minishing discharge rate. This is caused by the redox reaction occurred on the electrode, which can be represented as pseudo- capacitance. Nevertheless, in the 20th discharge process, the high potential range for the redox reaction is diminished by 0.25 V, and the pseudocapacitance also decreases. The 200th discharge curve is close to ideal one, for the redox process died away in high potential area, which is in accord with the vanishment of redox peaks in Fig.4.
It is inferred that the reaction occurred on the electrode is the redox process Mn(Ⅳ)
Mn(Ⅲ). At the same time, a few formed MnOOH combines with MnO2 irreversibly. But when discharging more deeply, the crystal lattices are destroyed, leading to irreversible electrode processes. As a result, the electrode resistance and the reaction resistance increase. The reactions could be considered as[13]
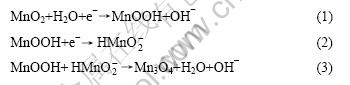
3.5 Equivalent series resistance and capacitance
The capacitance of electrode and equivalent series resistance can be calculated by[14]
C=4It/(mV) (4)
RESR=?V/I (5)
where C is the capacitance of electrode, F/g; I is the discharge current, A; t is the discharge time, s; m is the mass of active material, g; V is the discharge voltage, V. RESR is the equivalent series resistance of supercapacitor, Ω; ?V is the initial transitory voltage dispersion, V. The results are shown in Figs.7 and 8, respectively.
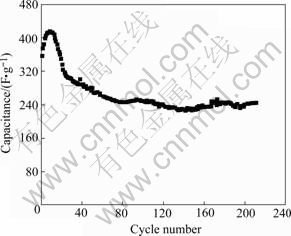
Fig.7 Discharge capacitance of MnO2 electrode
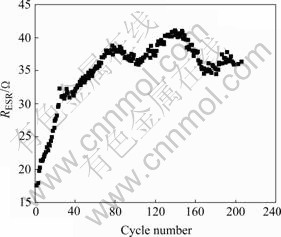
Fig.8 RESR of MnO2 supercapacitor
It can be found that during the first 40 charge- discharge cycles, the maximum capacitance of electrode is 416 F/g, declining 4.5 F/g per cycle; the minimum RESR is 17 Ω, ascending 0.36 Ω per cycle. This is mainly caused by the formation of Mn3O4 (Figs.2 and 4). Whereas, the capacitance tends to be steady during the next 160 cycles: the capacitance declines 0.3 F/g per cycle, reaches 240 F/g or so finally, while the RESR ascends 0.06 Ω per cycle, reaches about 36 Ω at the end.
4 Conclusions
1) The supercapacitors are of various capacitance characteristics in different discharge potential ranges, namely showing electrochemistry double layer capability in low potential range and pseudocapacitance in high potential range.
2) The electrochemistry inert Mn3O4 is mainly formed during the first 40 charge-discharge cycles, which makes the redox peaks in cyclic voltammograms disappear, and reduces the pseudocapacitance in high potential scope.
3) The maximum capacitance of capacitor electrode is 416 F/g and retains 240 F/g after 200 cycles; the RESR increases from 17 to 41 Ω during the charge-discharge cycles in 1.2 V discharge window at 200 mA/g.
References
[1] ANDREW B. Ultracapacitors: Why, how, and where is the technology [J]. Journal of Power Sources, 2000, 91: 37-50.
[2] WANG Yong-gang, ZHANG Xiao-gang. Preparation and electrochemical capacitance of RuO2/TiO2 nanotubes composites [J]. Electrochimica Acta, 2004, 49: 1957-1962.
[3] CHUNAN U, JAMS A R, BRANKON P. Characterization of sol-gel derived cobalt oxide xerogels as electrochemical capacitors [J]. Journal of the Electrochemical Society, 1998, 145(12): 4097-4102.
[4] MARTIN W, RALPH J B. What are batteries, fuel cells, and supercapacitors? [J]. Chem Rev, 2004, 104: 4246-4369.
[5] LIU X M, FUA S Y, HUANG C J. Synthesis, characterization and magnetic properties of β-MnO2 nanorods [J]. Powder Technology, 2005, 154(2/3): 120-124.
[6] VENKAT S, JOHN W W. An electrochemical route for making porous nickel oxide electrochemical capacitors [J]. Journal of the Electrochemical Society, 1997, 144(8): L210-L213.
[7] SUBRMANIAN V, ZHU H W, ROBERT V, AJAYAN P M, BING Q W. Hydrothermal synthesis and pseudocapacitance properties of MnO2 nanostructures [J]. J Phys Chem B, 2005, 109: 20207-20214.
[8] WANG X Y, WAN G, HUANG W G, SCBASTIAN P J, GAMBOA S. Sol–gel template synthesis of highly ordered MnO2 nanowire arrays [J]. Journal of Power Sources, 2005, 140: 211-215.
[9] CHANG J K, TSAL W T. Material characterization and electro- chemical performance of hydrous manganese oxide electrodes for use in electrochemical pseudocapacitors [J]. Journal of the Electrochemical Society, 2003, 150(10): A1333-A1338.
[10] FENG Yang-liu, ZHANG Mi-lin, CHEN Ye. Inorganic salt water solution reaction synthesis and capacitor characteristics of nano-MnO2 power [J]. Journal of the Chinese Ceramic Society, 2005, 33(3): 404-407. (in Chinese)
[11] LIU Li-qing, WANG Jian-ming, WU Mei-yin, FAN Yu-kai, ZHANG Jian-qing. Physical properties and electrochemical performance of γ-MnO2 with utrafine power [J]. Journal of Functional Materials, 2005, 36(3): 404-407. (in Chinese)
[12] YANG Hua-bin, ZHANG Yun-shi, ZHOU Zuo-xiang, JIN Ping-wei. Electrochemical impedance spectra study of the hyddrogen storage electrodep [J]. Journal of Alloys and Compounds, 1995, 231: 625-630.
[13] DENG Mei-gen, ZHANG Zhi-an, HU Yong-da. Study on carbon nanotubes/manganese dioxide composite electrode materials for supercapacitors [J]. Journal of the Chinese Ceramic Society, 2004, 32(4): 411-415. (in Chinese)
[14] ZHANG Li, ZOU Ji-yan, GUO Ying, WANG Quan-shui. Study on 40 V hybrid supercapacitor unit [J]. Acta Electronica Sinica, 2004, 32(8): 1253-1255. (in Chinese)
Corresponding author: LIU Kai-yu; Tel: +86-731-8660309; E-mail: kaiyuliu@263.net
(Edited by CHEN Wei-ping)