J. Cent. South Univ. (2017) 24: 515-520
DOI: 10.1007/s11771-017-3454-4

Characterization of hot deformation behavior of Al-Zn-Mg-Mn-Zr alloy during compression at elevated temperature
YAN Jie(严杰)1, PAN Qing-lin(潘清林)1, ZHANG Xiang-kai(张祥凯), SUN Xue(孙雪)1,
LI An-de(李安德)2, ZHOU Xun(周勋)2
1. School of Materials Science and Engineering, Central South University, Changsha 410083, China;
2. Suntown Technology Group Co., Ltd., Changsha 410200, China
Central South University Press and Springer-Verlag Berlin Heidelberg 2017
Abstract: The hot deformation behavior of Al-6.2Zn-0.70Mg-0.30Mn-0.17Zr alloy and its microstructural evolution were investigated by isothermal compression test in the deformation temperature range between 623 and 773 K and the strain rate range between 0.01 and 20 s-1. The results show that the flow stress decreased with decreasing strain rate and increasing deformation temperature. At low deformation temperature (≤673 K) and high strain rate (≥1 s-1), the main flow softening was caused by dynamic recovery; conversely, at higher deformation temperature and lower strain rate, the main flow softening was caused by dynamic recrystallization. Moreover, the slipping mechanism transformed from dislocation glide to grain boundary sliding with increasing the deformation temperature and decreasing the strain rate. According to TEM observation, numerous Al3Zr particles precipitated in matrix, which could effectively inhibit the dynamic recrystallization of the alloy. Based on the processing map, the optimum processing conditions for experimental alloy were in deformation temperature range from 730 K to 773 K and strain rate range from 0.033 s-1 to 0.18 s-1 with the maximum efficiency of 39%.
Key words: aluminum alloy; hot deformation; TEM; dynamic recrystallization; processing map
1 Introduction
As one of low Cu-containing aluminum alloys in 7xxx series, the Al-Zn-Mg-Mn alloy has an excellent combination of properties such as strong corrosion resistance and relatively high specific strength, which has been widely used for the components of high speed train [1-4]. The microstructures which are determined by deformation conditions have a great influence on macro- performance of aluminum profiles [5-7]. Therefore, researching the relationship between processing parameters and microstructural evolution is of great importance during optimizing the alloy’s thermo- mechanical process.
The flow stress, deformation temperature and strain rate are three basic parameters for characterizing hot working processes of alloys. The flow softening mechanisms, including dynamic recovery (DRV) and dynamic recrystallization (DRX), are closely associated with these processing parameters, leading to different kind of microstructures [8-10]. ZHANG et al [11] investigated the hot deformation behavior of carbon Cr-Ni-Mo alloyed steel and reported that DRX was likely to occur at higher temperatures and lower strain rates during hot deformation. But SHI et al [6] found that the softening mechanism of the 7150 aluminum alloy during hot compression was mainly dynamic recovery. Since alleys’ microstructure is directly dicided by processing conditions, to obtain some excellent alloy, we need to precisely control the processing parameters during hot deformation to obtain satisfied microstructure of alloys. Furthermore, the processing maps, based on dynamic material model (DMM), are being widely used to optimize the hot working conditions of the steels [11], titanium alloys [12], copper alloys [13], magnesium alloys [14], and aluminum alloys [15, 16]. Unfortunately, although the Al-6.2Zn-0.70Mg-0.30Mn-0.17Zr alloy has been used in industry, little research work has been focused on its microstructural evolution during hot deformation.
Accordingly, the goal of this work was to investigate the relationship between microstructural evolution and hot deformation parameters of Al-6.2Zn- 0.70Mg-0.30Mn-0.17Zr alloy during hot compressive deformation. The processing map was constructed in order to optimize the hot working conditions.
2 Experimental procedures and processing map principles
2.1 Experimental procedures
The material studied in the present work was Al-6.2Zn-0.70Mg-0.30Mn-0.17Zr alloy produced by semi-continuous casting. The chemical composition (mass fraction, %) of examined alloy is as follows: 6.2Zn, 0.7Mg, 0.3Mn, 0.17Zr, 0.15Cu, 0.1Cr, 0.2Ti, 0.28Fe, 0.32Si and the balanced Al. The ingot was homogenized at 738 K for 24 h, and then slowly cooled to room temperature in the air. Figure 1 shows the original grain structure of the experimental alloy. As can be seen from Fig. 1, the initial alloy consisted of equixed grain structure with the sizes on the order of 90 μm.
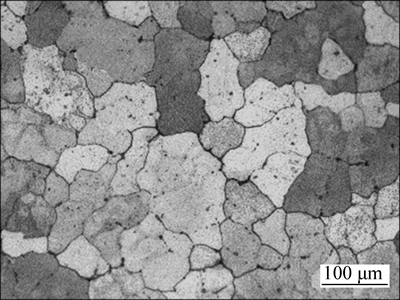
Fig. 1 Initial microstructure of experimental alloy
The hot compression tests were carried out on a Gleeble–3500 testing system. The strain rates were 0.01, 0.1, 1, 10 and 20 s-1 and the deformation temperatures were 623, 673, 723 and 773 K in the experiments. Cylindrical specimens were prepared according to ASTM E209 standard [17]. Before the compression tests, the specimens were heated to deformation temperature with the rate of 10 K/s, then held for 3 min at isothermal conditions, and afterwards deformed to a true strain of 0.7. When the deformation test finished, the specimens were quenched in cold water immediately in order to squeeze the hot-deformed microstructure for microstructural observation. The microstructure was observed using Leica metallographic microscope, FEI QUANTA-200 scanning electron microscope as well as TECNAI G220 transmission electron microscope. The examined specimens were prepared according to conventional methods [15].
2.2 Processing map principles
The principle of processing map was based on the dynamic materials model (DMM) developed by PRASAD et al [18, 19]. This model was widely used to study the plastic deformation of metal materials at high temperature. In this model, the materials under elevated temperature deformation conditions were considered to be a power dissipater, which could be represented by the strain rate sensitivity parameter m:
(1)
The microstructure evolution during the hot deformation was closely related to the efficiency of power dissipation, which was a dimensionless parameter defined as
(2)
Based on the principle of maximum rate of entropy production, an instability criterion was used to identify the flow instability regimes during hot deformation. The instability parameter
was defined as follows:
(3)
When parameters
was negative under a certain deformation condition, the metallurgical instability took place. Therefore, a processing map could be built by combining the instability map and the power dissipation map.
3 Results and discussion
3.1 True stress–true strain curves
Figure 2 shows the true stress–true strain curves obtained from experiment tests at some deformation conditions, and the values of steady state flow stress under different deformation conditions are given in Table 1. As can be seen in Fig. 2 and Table 1, the flow stress increased promptly with the increase of strain, and the steady state flow stress increased with the increase of strain rate or the decrease of deformation temperature.
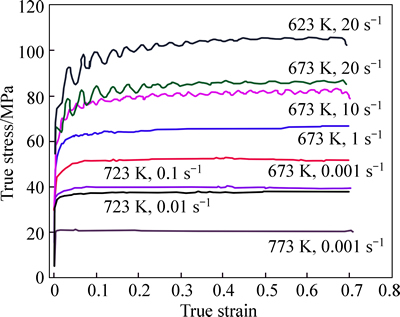
Fig. 2 True stress–true strain curves of alloy under some deformation conditions
Table 1 Steady state flow stress (MPa) under different deformation conditions
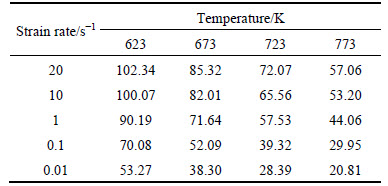
Generally, during aluminum alloys’ hot deformation process, work hardening, dynamic recovery as well as dynamic recrystallization are three common metallurgical phenomena, leading to the complex microstructural evolution [9]. At the early stage of the compression test, dislocation density in aluminum alloy increased sharply, causing the fast increase of flow stress. Work hardening phenomenon took place during this period. As deformation proceeded, the stored energy in deformed grains and grain boundaries was promptly accumulated, providing sufficient driving force for dislocation annihilation and recombination in subgrain boundaries. The dynamic recovery phenomenon occurred at this time, leading to the formation of subgrains and the ultimately stabilized level of dislocation density. Therefore, the work hardening and flow softening reached to a dynamic balance and flow stress could not increase despite the continuous increase of strain. When the flow stress reached to a steady state, the mobility of dislocation at higher temperature was enhanced. Meanwhile, there was sufficient time for dislocation reaction, when specimens were deformed at lower strain rate [9, 20]. The subgrains and the dynamic recrystallized nucleus were easily formed at higher temperature and lower strain rate. Consequently, the steady state flow stress was lower when specimen was deformed at higher temperature and lower strain rate.
3.2 Processing map
The processing map of experiment alloy at true strain of 0.65 is shown in Fig. 3. The contours on processing maps represent constant efficiency (%) of power dissipation, and shadow domains represent the instability domains. According to the previous study, the deformation mechanisms of the safe domains include DRV, DRX and super-plasticity. The energy consumption during the process of DRX is higher than that of DRV, and when the efficiency value is higher than critical efficiency value (0.30), the deformation mechanism is DRV [7, 16].
As can be seen from Fig. 3, there is a domain with high efficiency. The region with a higher efficiency usually corresponds to the optimum processingconditions [19]. In the present study, considering the instability map, the optimum processing conditions were in deformation temperature range from 730 K to 773 K and strain rate range from 0.033 s-1 to 0.18 s-1 with the maximum efficiency of 39%. The further analysis will be taken in the section of microstructural observation.
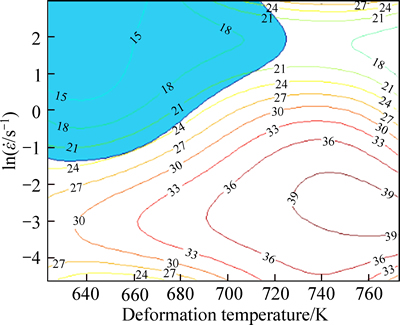
Fig. 3 Processing map of experimental alloy at strain of 0.65
3.3 Microstructure evolution
Typical metallographic graphs of the alloy under different deformation conditions are shown in Fig. 4. The microstructural evolution was strongly associated with deformation temperature and strain rate. As shown in Figs. 4(a)-(c), the initial grains were elongated and the deformed grain boundaries presented serrated features, when the experimental alloy deformed at a relatively low temperature (≤673 K) and high strain rate (≥1 s-1). However, the deformed microstructure became more homogeneous at higher deformation temperature and lower deformation strain rate (Fig. 4(d), with 723 K, 0.1 s-1). This is because more slip systems were activated at this deformation condition [9]. When the deformation temperature further increased, as shown in Fig. 4(e), a small amount of DRX grains could be observed, and some of the deformed grains kept their aspect ratios, but the sizes of these deformed grains were significantly larger than that of the DRX grains. In order to better analyze the deformation mechanism and microstructure evolution of the alloy under different deformation conditions, the specimens were observed by scanning electron microscope (SEM) at higher magnification, and the result was shown in Fig. 5. It can be easily found that the deformation bands were perpendicular distributed to the compress direction, when the specimen was deformed at lower temperature (Fig. 5(a)). Then the number of deformation bands decreased with the increase of temperature (Fig. 5(b)), and it eventually disappeared when the deformation temperature increased to 723K (Fig. 5(c)).
Based on the analysis of Figs. 4 and 5, it is suggested that the slipping mechanism of the experimental alloy during hot deformation changed from dislocation glide (DG) to grain boundary sliding (GBS) when the deformation temperature was high and strain rate was low, and this was in accordance with the result reported by VERLINDEN et al [22]; meanwhile, the results indicate that the dynamic recrystallization phenomenon occurred at higher deformation temperature and lower strain rate. These can be viewed from the following two points. On one hand, as described earlier (Section 3.1), it was easier to form subgrains and dynamic recrystallized nucleus when the specimen was deformed at higher temperature and lower strain rate. In addition, the mobility of grain boundaries was improved, which was beneficial to the growth of DRX grains. On the other hand, serving as a dominant deformation mechanism, grain boundary sliding (GBS) could take place along the deformed grain boundaries at higher deformation temperature and lower strain rate. Finer grains nearby the deformed grain boundary were easily formed because of the additional inhomogeneous strains of these places [9, 19, 21, 22]. Therefore, these finer grains could provide more nucleation sites, and the additional inhomogeneous strains were beneficial to further adding the driving force of dynamic recrystallization.
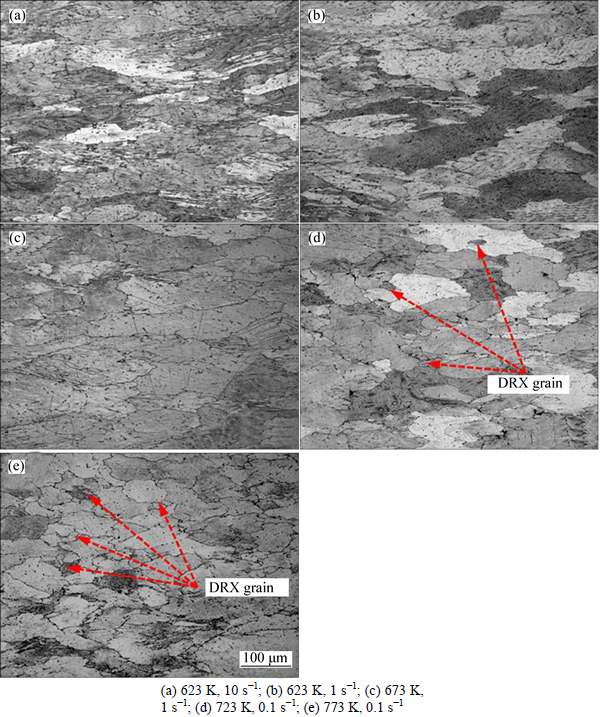
Fig. 4 Optical metallographs of specimens deformed under different conditions:
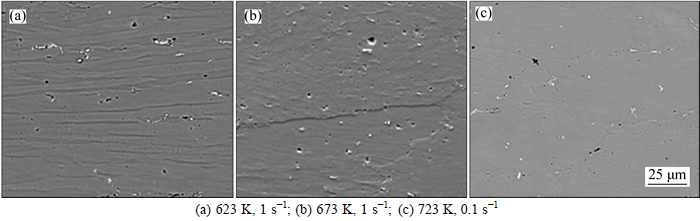
Fig. 5 SEM image of deformation microstructure of alloy under different deformation conditions:
Figure 6 shows the transmission electron micrographs (TEM) of deformed specimens under different deformation conditions. As can be seen from Fig. 6(a), high density of dislocations tangles and some curved subgrain boundaries were formed in specimen, when specimen was deformed at temperature of 623 K and strain rate of 10 s-1. Then the dislocation density decreased and the subgrain boundaries became straight with the decrease of strain rate (Fig. 6(b) with 623 K, 1 s-1). When the deformation temperature increased and strain rate decreased further, the subgrain size increased and large angle grain boundaries formed (Fig. 6(c) with 673 K, 1 s-1 and Fig. 6(d) with 723 K, 0.1 s-1). These were related to the enhancement of dislocations annihilate and cross-slip when specimen was deformed at higher temperature and lower strain rate. Figures 6(e) and 6(f) show a bright-field and a dark-field TEM micrographs taken from [001] direction of α(Al) matrix, respectively. As shown in dark-field TEM micrograph, it is obvious that the nano-scaled and fine spherical particles precipitated in α(Al) matrix. These fine spherical particles were confirmed by SAD pattern. As shown in Fig. 6(g), the diffraction spots distinguished from α(Al) matrix were Al3Zr particles with an Ll2 cubic crystal structure, which have been verified by SHA et al [23] who studied the early-stage precipitation in Al-Zn- Mg-Cu-Zr alloy. These coherent Al3Zr particles could pin dislocations and grain boundaries inα(Al) matrix, resulting in an inhibition of dynamic recrystallization during the hot working process.
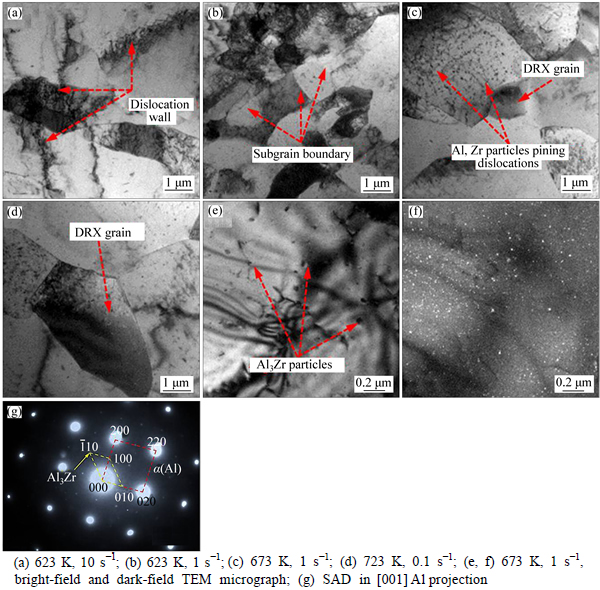
Fig. 6 TEM microstructures of alloy under different deformation conditions:
4 Conclusions
1) Deformation conditions had a significantly influence on the flow stress of experimental alloy during hot deformation. The flow stress decreased with the increase of deformation temperature and decrease of strain rate.
2) The main dynamic flow softening mechanisms of the alloy during hot deformation were considered as dynamic recovery and dynamic recrystallization. At low deformation temperature (≤673 K) and high strain rate (≥1 s-1), the main soften mechanism was dynamic recovery; conversely, at higher deformation temperature and lower strain rate, the main soften was dynamic recrystallization. The slipping mechanism transformed from dislocation glide (DG) to grain boundary sliding (GBS) with the increase of deformation temperature and the decrease of strain rate.
3) The optimum processing conditions for experiment alloy were in deformation temperature range from 730 K to 773 K and strain rate range from 0.033 s-1 to 0.18 s-1 with the maximum efficiency of 39%.
4) Numerous Al3Zr particles precipitated in matrix, which could effectively inhibit the dynamic recrystallization of the alloy.
References
[1] WILLIAMS J C, Jr STARKE E A. Progress in structural materials for aerospace systems [J]. Acta Mater, 2003, 51: 5775–5799.
[2] DENG Y, YIN Z M, ZHAO K, DUAN J Q, HE Z B. Effects of Sc and Zr microalloying additions on the microstructure and mechanical properties of new Al–Zn–Mg alloys [J]. J Alloys Compd, 2012, 530: 71–80.
[3] MENG Q J, FRANKEL G S. Effect of Cu content on corrosion behavior of 7xxx series aluminum alloys [J]. J Electrochem Soc, 2004, 151: B271-B283.
[4] CHEN L, ZHAO G Q, YU J Q, ZHANG W D. Constitutive analysis of homogenized 7005 aluminum alloy at evaluated temperature for extrusion process [J]. Mater Des, 2015, 66: 129–136.
[5] WLOKA J, HACK T, VIRTANEN S. Influence of temper and surface condition on the exfoliation behaviour of high strength Al–Zn– Mg–Cu alloys [J]. Corros Sci, 2007, 49: 1437–1449.
[6] SHI C J, MAO W M, CHEN X G. Evolution of activation energy during hot deformation of AA7150 aluminum alloy [J]. Mater Sci Eng A, 2013, 571: 83–91.
[7] MCQUEEN H J, JONAS J J. Plastic deformation of materials [M]. New York, Academic Press, 1975.
[8] MEYERS M A, CHAWLA K K. Mechanical behavior of materials [M]. New York, Cambridge University Press, 2009.
[9] SAKAI T , BELYAKOV A , KAIBYSHEV R , MIURA H , JONAS J J. Dynamic and post-dynamic recrystallization under hot, cold and severe plastic deformation conditions [J]. Prog Mater Sci, 2014, 60: 130–207.
[10] SAKAI T, MIURA H, GOLOBORODKOA A, SITDIKOVA O. Continuous dynamic recrystallization during the transient severe deformation of aluminum alloy 7475 [J]. Acta Mater, 2009, 57: 153–162.
[11] ZHANG C, ZHANG L W, SHEN W F, LIU C R, XIA Y N, LI R Q. Study on constitutive modeling and processing maps for hot deformation [J]. Mater Des, 2016, 90: 804–814.
[12] LIU G F, ZHANG S Z, CHEN L Q. Hot deformation behavior of Ti-6.5Al-3.5Mo-1.5Zr-0.3Si alloy with acicular microstructure [J]. J Cent South Univ, 2011, 18: 296-302
[13] WANG M H, HUANG L, CHEN M L, WANG Y L. Processing map and hot working mechanisms of Cu-Ag alloy in hot compression process [J]. J Cent South Univ, 2015, 22: 821-828
[14] WANG S Y. GAO L, LUO A A, LI D J, ZENG X Q. Hot deformation behavior and workability of pre-extruded ZK60A magnesium alloy [J]. Trans Nonferrous Met Soc China, 2015, 25: 1822–1830.
[15] LI B, PAN Q L, ZHANG Z Y, ZHOU J. Research on the hot deformation behavior of Al-Zn-Mg-Sc-Zr alloy during compression at elevated temperature [J]. J Mater Eng Perform, 2013, 22: 536–540.
[16] CHEN S Y, CHEN K H, PENG G S, CHEN X H, CEN Q H. Effect of heat treatment on hot deformation behavior and microstructure evolution of 7085 aluminum alloy [J]. J Alloys Compd, 2012, 537: 338–345.
[17] ASTM E209. Standard practice for compression tests of metallic materials at elevated temperatures with conventional or rapid heating rates and strain rates [S]. United States: Annual Book of ASTM Standards, 2010.
[18] PRASAD Y V R K, GEGEL H L, DORAIVELU S M, MALAS J C, MORGAN J T, LARK K A, BARKER D R. Modeling of dynamic material behavior in hot deformation: Forging of Ti-6242 [J]. Metall Mater Trans A, 1984, 15: 1883-1892.
[19] PRASAD Y V R K. Hot Working Guide: A compendium of processing maps [M]. ASM International, 1997.
[20] LI B, PAN Q L, YIN Z M. Microstructural evolution and constitutive relationship of Al–Zn–Mg alloy containing small amount of Sc and Zr during hot deformation based on Arrhenius-type and artificial neural network models [J]. J Alloys Compd, 2014, 584: 406–416.
[21] SAKAI T, YANG X Y, MIURA H. Dynamic evolution of fine grained structure and superplasticity of 7075 aluminum alloy [J]. Mater Sci Eng A, 1997, 234: 857–860.
[22] VERLINDEN B, DRIVER J, SAMAJDAR I, DOHERTY D. Thermo-mechanical processing of metallic materials [M]. Elsevier, 2007.
[23] SHA G, CEREZO A. Early-stage precipitation in Al–Zn–Mg–Cu alloy (7050) [J]. Acta Mater, 2004, 52: 4503–4516.
(Edited by YANG Bing)
Cite this article as: YAN Jie, PAN Qing-lin, ZHANG Xiang-kai, SUN Xue, LI An-de, ZHOU Xun. Characterization of hot deformation behavior of Al-Zn-Mg-Mn-Zr alloy during compression at elevated temperature [J]. Journal of Central South University, 2017, 24(3): 515-520. DOI: 10.1007/s11771-017-3454-4.
Foundation item: Project(2016GK1004) supported by the Science and Technology Major Project of Hunan Province, China
Received date: 2016-01-08; Accepted date: 2016-03-29
Corresponding author: PAN Qing-lin, Professor; Tel: +86-731-88830933; E-mail: pqlmta102@163.com