Trans. Nonferrous Met. Soc. China 24(2014) 1562-1570
Differential utilization of cyclic, orthorhombic α- and chain-like polymeric μ-sulfur by Acidithiobacillus ferrooxidans
An-an PENG1,2, Jin-lan XIA1,2, Hong-chang LIU1,2, Zhen-yuan NIE1,2, Yi YANG1,2, Wei ZHU1,2
1. School of Minerals Processing and Bioengineering, Central South University, Changsha 410083, China;
2. Key Laboratory of Biometallurgy of Ministry of Education, Central South University, Changsha 410083, China
Received 20 May 2013; accepted 10 September 2013
Abstract: The differential utilization of cyclic, orthorhombic α-sulfur (α-S) and chain-like polymeric μ-sulfur (μ-S) by Acidithiobacillus ferrooxidans was investigated. The growth and sulfur oxidation results indicated that utilization of μ-S by A. ferrooxidans was clearly different from α-S. Even if the planktonic cells were produced, the fall of pH and the rise of sulfate concentration were the same after 300 h on each substrate, the speeds of the planktonic cells increase, pH decrease and sulfate concentration increase in the earlier cultivation stage were faster on polymeric sulfur compared with the orthorhombic form. The adsorption capacity of the cells was higher on μ-S than on α-S. The results of SEM, DRIFTS and XRD analyses indicated that the surfaces of α-S and μ-S were modified differently by cells. Differential expression of 11 selected sulfur adsorption-activation and metabolism relevant genes was detected by RT-qPCR. The results showed that the expression of the hydrophobic substrate transport proteins and the sulfur metabolism related proteins was up-regulated, and the adsorption and activation related proteins were down-regulated when the cells were grown on μ-S, suggesting that μ-S could be more easily bio-adapted and activated than α-S.
Key words: sulfur oxidation; Acidithiobacillus ferrooxidans; α-sulfur; μ-sulfur; RT-qPCR
1 Introduction
The formation and oxidation of elemental sulfur is considered an important part of many industrial operations, such as hydrometallurgy and sulfate- containing wastewater treatment [1,2]. In bioleaching process, metal sulfides are dissolved by chemical attack of iron(III) ions and/or protons, in the presence of sulfur-oxidizing bacteria (SOB) [3]. Elemental sulfur may accumulate on the mineral surface as the main component of passivation layer during the minerals dissolution [4]. It is known that SOB contribute to eliminate the sulfur layer, which oxidizes sulfur and other intermediary sulfur compounds into sulfuric acid, and generates the protons [5].
Many studies have mentioned the production of biological sulfur by SOB in the oxidation of sulfide to sulfate or other sulfur compounds [6]. Actually, sulfur produced by microorganisms can be stored as sulfur globules, located either inside or outside the cells. The sulfur globules maybe consist of long sulfur chains terminated with organic groups, sulfur rings (S8), polysulfides or polythionates, etc. [6,7]. Biologically- produced sulfur can be oxidized faster than sulfur flower (mainly S8) by Acidithiobacillus species, resulting in higher rates of sulfuric acid production and metal dissolution [2,8].
The study of the metabolism of sulfur is complicated because of the multiple oxidation state of sulfur from -2 to +6 valence states. Several enzymes have been identified for sulfur oxidation of SOB. However, the sulfur oxidation model is not clearly established even for the most studied SOB, Acidithiobacillus ferrooxidans [9]. In most cases, an activation reaction prior to bio-oxidation is postulated, because of the extreme hydrophobicity of elemental sulfur. This activation reaction could include the break of S8 ring by the extracellular polymeric substance (EPS), resulting in the formation of linear inorganic or organic polysulfanes [10]. Then the sulfane sulfur is transported into the periplasmic space and oxidized to sulfate ultimately by the enzyme system [10,11].
Elemental sulfur can exist in a variety of allotropic forms, and it is mainly a mixture of cyclo-octa sulfur (α-S) and chain-like polymeric sulfur (μ-S). Cyclic, orthorhombic α-S, is the predominant allotrope found in nature, which contains puckered rings of S8 [12]. The bonding energy between S—S bonds in μ-S is 2.4 kJ/mol weaker than in α-S, therefore, μ-S might be more accessible than α-S for SOB [12,13].
There are only a few studies focusing on evaluation of the ability and preference of bacteria to oxidize specific sulfur allotropes. LAISHELY et al [14] suggested that the oxidation of sulfur by A. albertis could be affected by particle size, specific surface area, and the molecular microcrystalline composition of the sulfur, but there was no significant difference in the oxidation rates of α-S and μ-S. FRANZ et al [15] reported that phototrophic sulfur bacteria preferentially used the chain-like μ-S. BLIGHT et al [16] observed that a heterogeneous culture of SOB preferentially utilized the orthorhombic α-S rather than polymeric μ-S. HE et al [17] found that α-S possibly was converted to μ-S before oxidation by A. ferrooxidans. Therefore, the preferential utilization of the sulfur allotropes by SOB is in dispute.
In this study, the differential utilization of α- and μ-sulfur by A. ferrooxidans was investigated. Analysis of the surface characteristics of both cells and sulfur allotropes and the relative expression levels of sulfur metabolism related-genes were also presented. The results are helpful for elucidating the preferential use of sulfur allotropes and the sulfur activation-oxidation mechanism by A. ferrooxidans during the bioleaching process.
2 Experimental
2.1 Pretreatment of sulfur allotropes
The powder samples of α-S and μ-S were pretreated according to the description by ZHANG et al [18], in order to remove the inorganic sulfides and organic matter from the elemental sulfur surface. Non-oil-treated polymeric μ-sulfur IS-60 (65.8% (w/w) of insoluble sulfur, purchased from Wuxi Huasheng Rubber Technical Co., Ltd., China) was continuously stirred in liquid CS2 (l g/mL, μ-S/CS2) for 20 min, then the CS2 phase was discarded. This process was repeated at least 5 times before vacuum drying. Sublimed sulfur (AR, purchased from Sinopharm Chemical Reagent Beijing Co., Ltd.) was sufficiently dissolved in the solvent CS2 (0.5 g/mL, α-S/CS2). The insoluble part was filtered off and the yellow solution was evaporated and crystallized in vacuum. The sulfur samples were ground to fine powder with the size of 75-38 mm and stored at 4 °C. The samples were characterized by the XRD and Raman spectra analyses. The tablets of the α-sulfur or μ-sulfur were made by a manual hydraulic tablet press under 9.8 MPa of pressure.
2.2 Strain and culture conditions
A. ferrooxidans strain ATCC 23270 was purchased from American Type Culture Collection (ATCC), stored and cultivated in the Key Laboratory of Biometallurgy of Ministry of Education, Central South University, Hunan province, China. A. ferrooxidans ATCC 23270 was cultured in 9K basal medium containing the following components: 0.5 g/L MgSO4·7H2O, 0.5 g/L K2HPO4, 3.0 g/L (NH4)2SO4, 0.1 g/L KCl, 0.01 g/L Ca(NO3)2, pH 2.0, with 44.2 g/L FeSO4·7H2O as the energy substrate. Then the active culture was inoculated to the liquid basal media with α-S (10 g/L) or μ-S (10 g/L) powder as the energy source, respectively. The initial cell number was 8×106 mL-1. A. ferrooxidans was incubated in 250 mL Erlenmeyer flask containing 100 mL medium on an air bath rotary shaker at 30 °C and 170 r/min. The bacteria grown on the tablet substrates were cultured under aeration at 30 °C. Ten tablets were bathed in 150 mL 9K basal media in an aeration culture apparatus with 8×106 mL-1 inoculated and 0.5 L/min aeration rate. They were picked up after 10 d incubation for surface observation.
2.3 Microbial adsorption measurements
The surface areas of the two sulfur allotropes were tested by nitrogen adsorption method using a surface area porosity analyzer (Quantachrome, America). The adsorption of bacteria to sulfur substrate was calculated by the difference between the number of initial inoculum and the number of bacteria remaining in the supernatant after 1 h.
2.4 Growth and sulfur oxidation determination
Cell growth was monitored by direct cell counting every 24 h. The pH of the culture medium was measured with a pH meter (pHs-25, E-201-C compound electrode), sulfate concentration was determined by barium sulfate turbidimetry [19] with the modification, and 12% ethanol and 0.004 g/L Tween-80 were used as the conditioning reagents (pH 1.0).
2.5 Characteristics of α-S and μ-S
The surface morphologies of the tablets of α-S and μ-S were observed by scanning electron microscope (NovaTM NanoSEM 230, FEI, USA) before and after the experiments with A. ferrooxidans.
The α-S and μ-S before and after the experiments with A. ferrooxidans ATCC 23270, and the cells grown on different elemental sulfur forms were thoroughly washed with di-distilled water and dried in vacuum, and then measured by diffuse reflectance infrared Fourier transform spectroscopy (DRIFTS) on Thermo Nicolet Nexus 670 FTIR Spectrometer in the range of 500-4000 cm-1 at step width of 2 cm-1. The X-ray diffraction (XRD) patterns of the powder samples were collected by an X-ray diffractometer (DX 2000, Dandong Fangyuan Instrument Co. LTD., China) with Cu Kα radiation (λ=0.154 nm). The Raman spectra were recorded at room temperature on a LABRAM-010 Confocal Laser Micro Raman spectrometer (HORIBA Jobin Yvon, French) with excitation of the samples by He-Ne laser (wavelength: 633 nm) at 1 s intervals.
2.6 Quantitative real-time PCR
The effects of different sulfur allotropes on the metabolism of the bacteria were analyzed by quantitative real-time PCR (RT-qPCR) analysis of eleven putative sulfur-activation relevant protein genes. These eleven genes encode three outer membrane proteins (our unpublished work on the sulfur oxidation related membrane proteins of A. ferrooxidans) and five extracellular proteins [20] according to our results of comparative proteomics study and three proteins annotated to play important roles during sulfur oxidation process [9,21]. 16S rRNA (afe_2854) was chosen as reference gene for RT-qPCR analysis. The selected genes with their annotated functions and primer sequences are listed in Table 1. The primer design, total RNA extraction, cDNA synthesization, and RT-qPCR were performed according to the previous description [22].
3 Results and discussion
3.1 Identification of sulfur allotropes
Raman spectroscopy and XRD analysis are useful to characterize the various allotropes of elemental sulfur. Both cyclic- and polymeric-sulfur absorb only in the near UV with a wing into the violet region of the visible spectrum [13]. The Raman spectra of the two allotropes are shown in Fig. 1 and the main peaks are well consistent with those in literatures [13,16]. The bands at 400-500 cm-1 and 150-250 cm-1 are assigned to S—S bonds stretching, and S—S—S bonds bending, respectively [23,24].
The peaks at 469.8 and 240.8 cm-1 and 257.8, 271.5 and 453 cm-1 represent the α-S8 and μ-S, respectively. The very weak signals at ca. 150 and 220 cm-1 on the spectrum of μ-S are probably caused by instability of the polymeric sulfur and the presence of S8.
Table 1 Primers for RT-qPCR detection of genes related to sulfur metabolism and reference gene (16S rRNA)
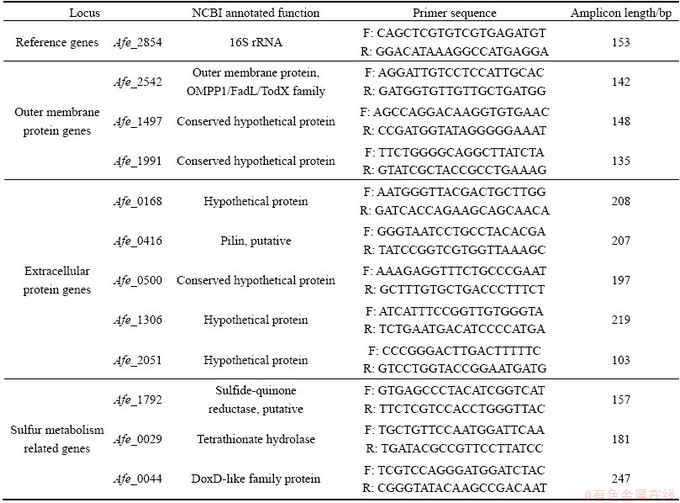
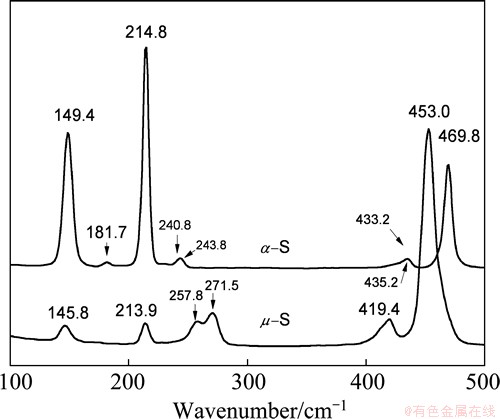
Fig. 1 Raman spectra of α- and μ- sulfur powder samples
3.2 Growth and sulfur oxidation of A. ferrooxidans grown on α-S and μ-S
The curves of growth, pH and sulfate concentration of A. ferrooxidans grown on α-S and μ-S powders are shown in Figs. 2(a)-(c), respectively. It can be found in Fig. 2(a) that the number of planktonic cells in the culture medium with μ-S was lower than that with α-S in the lag phase. A. ferrooxidans cultured with μ-S showed logarithmic growth after only 50 h while the bacteria cultured with α-S required at least 72 h before the exponential growth was observed. It was evident that the cell number of A. ferrooxidans grown on μ-S was higher than α-S between 100 h and 200 h. Accordingly, the concentrations of protons and sulfate (Figs. 2(b) and (c)) were also higher with μ-S than α-S, especially at the lag phase and the earlier logarithmic phase. But during 200-300 h, decrease of pH and the rate of sulfate production (d[SO4]/dt) was much faster in the experiment with α-S. No distinct difference in the number of planktonic cells was observed after the growth reached the stationary phase.
The results indicate that there might be different adaption features or chemotaxis response of A. ferrooxidans to the two sulfur allotropes, and cells probably adapted to μ-S faster. This is inconsistent with the results by BLIGHT et al [16], who showed that α-S was preferentially oxidized during batch culture. However, the mixed culture in their study might not include A. ferrooxidans, and a combined effect of several bacteria may also be different.
3.3 Adsorption behavior of A. ferrooxidans
The Lagergren pseudo first-order rate equation was used to model the A. ferrooxidans adsorption to the surface of α-S and μ-S powders [25,26]. The adsorption curves are shown in Fig. 3. The results show that the adsorption equilibriums were reached in about 40 min. The extent of adsorption in the μ-S media was clearly higher than that in the α-S media.
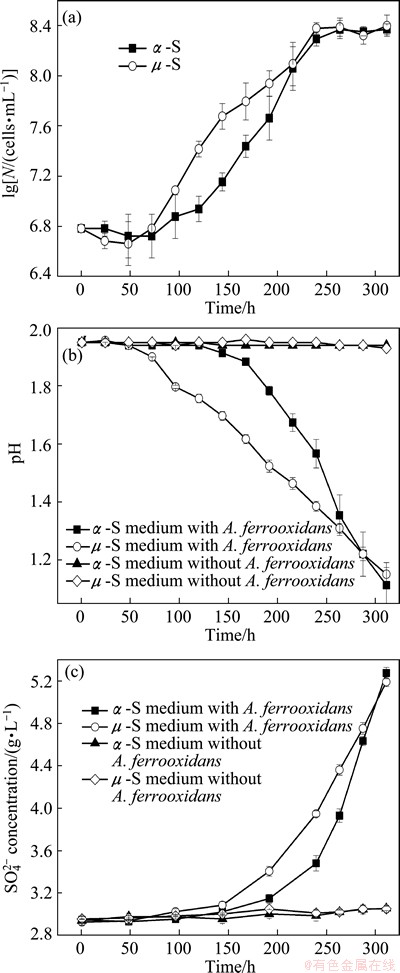
Fig. 2 Growth curves (a), pH curves (b) and sulfate concentration curves (c) of A. ferrooxidans cultured with α-S and μ-S
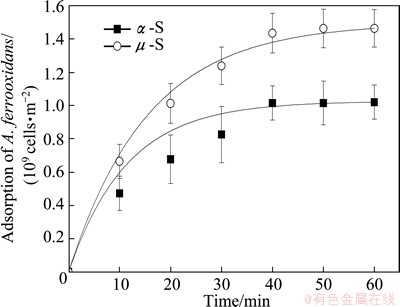
Fig. 3 Adsorption curves of A. ferrooxidans on α- or μ-S
3.4 Changes in surface morphology of α-S and μ-S after growing of A. ferrooxidans
It is known that elemental sulfur is chemically inert at normal temperature and pressure, and the sterile acidic media do not affect the surface of sulfur allotropes [27]. The changes of morphology of the orthorhombic α-S and polymeric μ-S oxidized by A. ferrooxidans are shown in Fig. 4. The results show that, in contrast to the originally smooth and clean surface of the orthorhombic α-S (Fig. 4(a)), the surface of α-S after 10 d was colonized by cells (Figs. 4(b) and (c)). The surface of μ-S also showed significant changes after bio-oxidation (Figs. 4(d) and (e)). It is evident that biofilms of cells were formed on the surface of μ-S (Fig. 4(f)).
3.5 DRIFTS analysis
Diffuse reflection infrared Fourier transform (DRIFT) spectra of α- and μ-S before and after growing with A. ferrooxidans, and the bacterial cells themselves grown on the two allotropes of sulfur are shown in Fig. 5. The spectra of the sulfur after treatment with A. ferrooxidans became much more complex due to the appearance of the absorption bands of organic compounds. The broad band at 3100-3500 cm-1 is assigned to —OH, and protein —NH— and —NH2 groups. The bands at 1630-1720 cm-1 and near 1540 cm-1 represent —C=O stretching and —NH2 vibration, respectively, and these two bands always suggest the presence of protein amide group (—CONH—) [28,29].
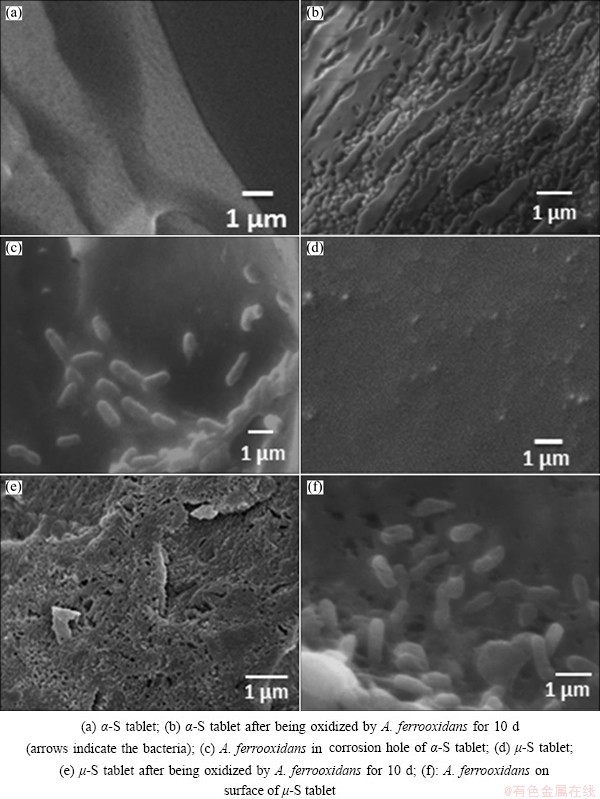
Fig. 4 Surface morphology of α-S tablet (a, b, c) and μ-S tablet (d, e, f) before and after growing with A. ferrooxidans ATCC 23270
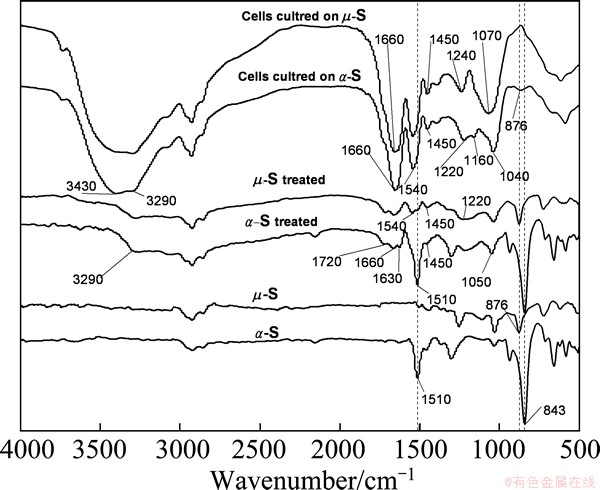
Fig. 5 DRIFT spectra of α-S and μ-S before and after growing with A. ferrooxidans, and cells grown on α-S and μ-S, respectively
The sharp bands near 1450 cm-1 characterize bending vibration of —CH3 and CH2 groups. The sharp bands near 1240 cm-1 and at 1040-1220 cm-1 represent stretching vibrations of C=S and S=O group, respectively. These results reveal that the surfaces of both α-S and μ-S were modified by the bacterial growth. The differences in the relative intensity and position of most absorbance bands at 1000-1700 cm-1 suggest the different chemical compositions of EPS excreted by the stimulation of the different types of energy substrates.
It is worthy to mention that the bands at 876, 843 cm-1 and 1510 cm-1 are significantly different in the spectra of the two allotropes of sulfur. The peaks at 843 cm-1 and 1510 cm-1 could be described as the characteristic of α-S, and the peak at 876 cm-1 probably is the characteristic of μ-S.
It is generally believed that the activation of sulfur occurs in the interface microenvironment filled with EPS between the attached cells and the substrate. In this microenvironment, there are abundant metabolic intermediates related to the activation of the elemental sulfur, for instance, polysulfide, sulfur-rich organic polysulfanes [10,11]. The band at 876 cm-1 was also observed in the spectra of the cells grown on α-S, indicating some polymeric sulfur-like structure formed during the assimilation of α-S. It seems to support that the sulfur ring of α-S was cleaved or activated firstly, and the preferable form used by A. ferrooxidans was polymeric μ-sulfur [17].
3.6 XRD analysis
The XRD spectra of α-S and μ-S before and after treatment by A. ferrooxidans are shown in Fig. 6. The XRD spectra of μ-S show amorphous characteristics, apparently comparable to that of the insoluble polymeric sulfur (PDF 20-1227) [30]. But the weak peaks found in the magnified spectra reveal a very small amount of other allotropes of sulfur, e.g., traces of S7 involved more or less because of the instability of this allotrope [12]. The spectra of α-S reflect the obvious orthorhombic structure and are corresponded closely with the PDF 8-247 of the International Centre for Diffraction Data. The alternative of the relative intensity indicates that the (026) and (040) planes of the orthorhombic crystal were preferentially damaged.
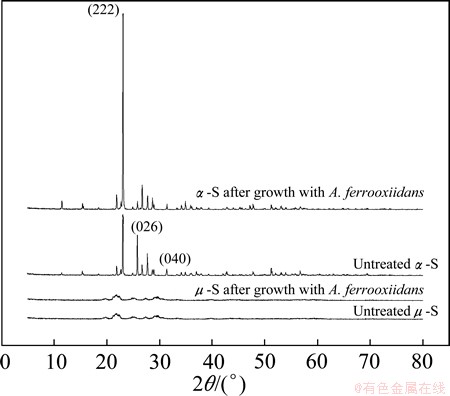
Fig. 6 XRD spectra of α-S and μ-S before and after growing with A. ferrooxidans
3.7 Differential expressions of sulfur metabolism relevant genes of A. ferrooxidans grown on α-S and μ-S
Table 2 shows the differential expressions of the selected genes between the cells grown on α-S and μ-S (in terms of lb[n(μ-S)/n(α-S)]. The results indicate that the structure of the allotropes has obvious effect on the expressions of certain functional genes.
Bacterial outer membrane proteins (OMPs) are integral membrane proteins that reside in the outer membrane of gram-negative bacteria, which perform a series of functions, for instance, adhesion, structure, efflux channel and enzyme. The gene Afe_2542 encodes FadL, an OMP responsible for the transport of hydrophobic substrates into the periplasm [31]. The gene Afe_1497 and Afe_1991 encode two conserved hypothetical proteins, and both of them have high degree of homology to the outer membrane porin protein (gi|198247860, NCBI) of A. ferrooxidans ATCC 53993. Porins are often the outer membrane channels relevant to the uptake of relatively water-soluble substrates. Compared with α-S, the expression of FadL protein Afe_2542 in the cells grown on μ-S was up-regulated and there were no significant changes in the expression level of the other two putative porin genes. This indicates that the bacteria grown on μ-S are more efficient in hydrophobic substrate transport than those grown on α-S.
Table 2 RT-qPCR expression data for relevant validated genes
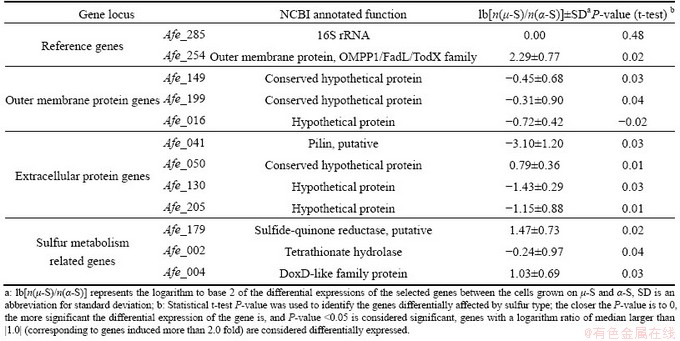
The selected extracellular proteins are cysteine-rich proteins, which were considered to be directly involved in the sulfur activation [20]. Down-regulation of the genes encoding the proteins Afe_0416, Afe_1306 and Afe_2051 in the cells grown on μ-S indicates the important role of the thiol groups of cysteine in α-S activation, and they may catalyze the transition of ring of S8 to —S—S— group [10]. Adhesion and biofilm formation are critical steps for colonization and subsequent sulfur assimilation. The putative pilin protein Afe_0416 is potentially involved in the formation of a type IV pilus, which is responsible for the secretion and adherence [21]. In this study, the gene Afe_0416 was significantly down-regulated in the cells grown on μ-S compared with α-S, indicating the important role of the pilin of A. ferrooxidans in adsorption and activation of α-S substrate. The chain-like μ-S seems to be easier to enable the adhesion of A. ferrooxidans.
The expressions of three typical sulfur metabolism related genes in the cells grown on the two allotropes of sulfur were also studied. Sulfide/quinone (Q) oxidoreductase (SQR) encoded by the gene Afe_1792 was suggested to catalyze the reaction, H2S+Q→H2Sn+QH2, and involved in the oxidation of sulfide to sulfur [32]. Tetrathionate hydrolase encoded by tetH (Afe_0029) catalyzes the disproportionation reaction, splitting
into other sulfur compounds, namely, S0,
and
. Tetrathionate hydrolase is thought to be a crucial enzyme because tetrathionate was detected as a key intermediate during sulfur oxidation in A. ferrooxidans [33]. DoxDA (Afe_0044) was predicted to encode a thiosulfate: quinone oxidoreductase (TQR) that splits thiosulfate into sulfur and sulfite [34]. Up-regulation of Afe_1792 and Afe_0044 indicates higher efficiency of electron transfer from sulfide to membrane-intrinsic quinones and quinone-binding mediated by SQR and the reaction catalyzed by TQR. It seems that A. ferrooxidans grown on μ-S has higher oxidation activity than on α-S.
4 Conclusions
1) The speeds of the planktonic cells increase, pH decrease and sulfate concentration increase in the earlier cultivation stage are faster for A. ferrooxidans grown on polymeric sulfur compared with the orthorhombic form.
2) A. ferrooxidans adapted to μ-S faster and more cells adsorbed onto μ-S than onto α-S.
3) The results of SEM, DEIFTS and XRD analyses indicate that the surface characteristics of sulfur are modified differently by cells. Both α-S and μ-S are colonized by A. ferrooxidans cells after 10 d cultivation. The chemical compositions of the EPS of A. ferrooxidans cells grown on α-S and μ-S are different. The (026) and (040) planes of the orthorhombic crystal are preferentially damaged by A. ferrooxidans.
4) The differential expressions of sulfur metabolism relevant genes suggest that μ-S could be more easily bio-adapted and activated than α-S.
References
[1] KLAUBER C. A critical review of the surface chemistry of acidic ferric sulphate dissolution of chalcopyrite with regards to hindered dissolution [J]. International Journal of Mineral Processing, 2008, 86(1-4): 1-17.
[2]
R, JANSSEN A, GROTENHUIS J T C, LETTINGA G, RULKENS W H. Possibilities for using biologically-produced sulphur for cultivation of Thiobacilli with respect to bioleaching processes [J]. Bioresource Technology, 1994, 48(3): 221-227.
[3] SAND W, GEHRKE T, JOZSA P G, SCHIPPERS A. (Bio)chemistry of bacterial leaching direct vs. indirect bioleaching [J]. Hydrometallurgy, 2001, 59(2-3): 159-175.
[4] FU K B, LIN H, MO X L, WANG H, WEN H W, WEN Z L. Comparative study on the passivation layers of copper sulphide minerals during bioleaching [J]. International Journal of Minerals, Metallurgy, and Materials, 2012, 19(10): 886-892.
[5] SCHIPPERS A, SAND W. Bacterial leaching of metal sulfides proceeds by two indirect mechanisms via thiosulfate or via polysulfides and sulfur [J]. Applied and Environmental Microbiology, 1999, 65(1): 319-321.
[6] KLEINJAN W E, KEIZER A, JANSSEN A J H. Biologically produced sulfur [J]. Topics in Current Chemistry, 2003, 230: 167-188.
[7] JANSSEN A J H, LETTINGA G, de KEIZER A. Removal of hydrogen sulphide from wastewater and waste gases by biological conversion to leemental sulphur colloidal and interfacial aspects of biologically produced sulphur particles [J]. Colloids and Surfaces A: Physicochemical and Engineering Aspects, 1999, 151: 389-398.
[8] SEIDEL H, WENNRICH R, HOFFMANN P,
C. Effect of different types of elemental sulfur on bioleaching of heavy metals from contaminated sediments [J]. Chemosphere, 2006, 62(9): 1444-1453.
[9] QUATRINI R, APPIA-AYME C, DENIS Y, JEDLICKI E, HOLMES D S, BONNEFOY V. Extending the models for iron and sulfur oxidation in the extreme Acidophile Acidithiobacillus ferrooxidans [J]. BMC Genomics, 2009, 10(1): 394.
[10] ROHWERDER T, SAND W. The sulfane sulfur of persulfides is the actual substrate of the sulfur-oxidizing enzymes from Acidithiobacillus and Acidiphilium spp [J]. Microbiology, 2003, 149(7): 1699-1710.
[11] GHOSH W, DAM B. Biochemistry and molecular biology of lithotrophic sulfur oxidation by taxonomically and ecologically diverse bacteria and archaea [J]. FEMS Microbiology Reviews, 2009, 33(6): 999-1043.
[12] MEYER B. Elemental sulfur [J]. Chemical Reviews, 1976, 76(3): 367-388.
[13] ECKERT B, STEUDEL R. Molecular spectra of sulfur molecules and solid sulfur allotropes [J]. Topics in Current Chemistry, 2003, 231: 31-98.
[14] LAISHELY E J, BRYANT R D, KORRYN B W, HYNE J B. Microcrystalline structure and surface area of elemental sulphur as factor influencing its oxidation by Thiobacillus albertis [J]. Canadian Journal of Microbiology, 1986, 32(3): 237-242.
[15] FRANZ B, LICHTENBERG H, DAHL C, HORMES J, PRANGE A. Utilization of 'elemental' sulfur by different phototrophic sulfur bacteria (Chromatiaceae, Ectothiorhodospiraceae): A sulfur K-edge XANES spectroscopy study [J]. Journal of Physics: Conference Series, 2009, 190(1): 012200.
[16] BLIGHT K R, CANDY R M, RALPH D E. The preferential oxidation of orthorhombic sulfur during batch culture [J]. Hydrometallurgy, 2009, 99(1-2): 100-104.
[17] HE H, XIA J L, HUANG G H, JIANG H C, TAO X X, ZHAO Y D, HE W. Analysis of the elemental sulfur bio-oxidation by Acidithiobacillus ferrooxidans with sulfur K-edge XANES [J]. World Journal of Microbiology and Biotechnology, 2010, 27(8): 1927-1931.
[18] ZHANG C G, XIA J L, ZHANG R Y, PENG A A, NIE Z Y, QIU G Z. Comparative study on effects of Tween-80 and sodium isobutyl-xanthate on growth and sulfur-oxidizing activities of Acidithiobacillus albertensis BY-05 [J]. Transactions of Nonferrous Metals Society of China, 2008, 18(4): 1003-1007.
[19] KOLMERT
, WIKSTR M P, HALLBERG K B. A fast and simple turbidimetric method for the determination of sulfate in sulfate-reducing bacterial cultures [J]. Journal of Microbiological Methods, 2000, 41(3): 179-184.
[20] ZHANG C G, ZHANG R Y, XIA J L, ZHANG Q, NIE Z Y. Sulfur activation-related extracellular proteins of Acidithiobacillus ferrooxidans [J]. Transactions of Nonferrous Metals Society of China, 2008, 18(6): 1398-1402.
[21]
J, PEDROSO I, QUATRINI R, DODSON R J, TETTELIN H, BLAKE R, EISEN J A, HOLMES D S. Acidithiobacillus ferrooxidans metabolism: from genome sequence to industrial applications [J]. BMC Genomics, 2008, 9(1): 597.
[22] PENG A A, LIU H C, NIE Z Y, XIA J L. Effect of surfactant Tween-80 on sulfur oxidation and expression of sulfur metabolism relevant genes of Acidithiobacillus ferrooxidans [J]. Transactions of Nonferrous Metals Society of China, 2012, 22(12): 3147-3155.
[23] KALAMPOUNIAS A G, KASTRISSIOS D T, YANNOPOULOS S N. Structure and vibrational modes of sulfur around the λ-transition and the glass-transition [J]. Journal of Non-Crystalline Solids, 2003, 326-327: 115-119.
[24] STEUDEL R, ECKERT B. Comment on “Probing the sulfur polymerization transition in situ with Raman spectroscopy” [J]. J Chem Phys, 2004, 121: 6573.
[25] HO Yuh-shan. Citation review of Lagergren kinetic rate equation on adsorption reactions [J]. Scientometrics, 2004, 59(1): 171-177.
[26] TAN S N, CHEN M. Early stage adsorption behaviour of Acidithiobacillus ferrooxidans on minerals I: An experimental approach [J]. Hydrometallurgy, 2012, 119-120: 87-94.
[27] BRIAND L E, BONETTO R D, LADAGA J L, DONATI E. Bulk and surface characterization of crystalline and plastic sulphur oxidized by two Thiobacillus species [J]. Process Biochemistry, 1999, 34(3): 249-256.
[28] GU G, SU L, CHEN M, SUN X, ZHOU H. Bio-leaching effects of Leptospirillum ferriphilum on the surface chemical properties of pyrite [J]. Mining Science and Technology, 2010, 20(2): 286-291.
[29] HE H, YANG Y, XIA J L, DING J N, ZHAO X J, NIE Z Y. Growth and surface properties of new thermoacidophilic Archaea strain Acidianus manzaensis YN-25 grown on different substrates [J]. Transactions of Nonferrous Metals Society of China, 2008, 18(6): 1374-1378.
[30] GELLER S. Pressure-induced phases of sulfur [J]. Science, 1966, 152(3722): 644-646.
[31] HEARN E M, PATEL D R, LEPORE B W, INDIC M, van den BERG B. Transmembrane passage of hydrophobic compounds through a protein channel wall [J]. Nature, 2009, 458(7236): 367-370.
[32] WAKAI S, TSUJITA M, KIKUMOTO M, MANCHUR M A, KANAO T, KAMIMURA K. Purification and characterization of sulfide:quinone oxidoreductase from an acidophilic iron-oxidizing bacterium, Acidithiobacillus ferrooxidans [J]. Bioscience, Biotechnology, and Biochemistry, 2007, 71(11): 2735-2742.
[33] KANAO T, KAMIMURA K, SUGIO T. Identification of a gene encoding a tetrathionate hydrolase in Acidithiobacillus ferrooxidans [J]. Journal of Biotechnology, 2007, 132(1): 16-22.
[34] LLER F H M, BANDEIRAS T M, URICH T, TEIXEIRA M, GOMES C M, KLETZIN A. Coupling of the pathway of sulphur oxidation to dioxygen reduction: Characterization of a novel membrane-bound thiosulphate:quinone oxidoreductase [J]. Molecular Microbiology, 2004, 53(4): 1147-1160.
嗜酸氧化亚铁硫杆菌对环状硫和线状硫的氧化利用差异
彭安安1,2,夏金兰1,2,刘红昌1,2,聂珍媛1,2,杨 益1,2,朱 薇1,2
1. 中南大学 资源加工与生物工程学院,长沙 410083;
2. 中南大学 生物冶金教育部重点实验室,长沙 410083
摘 要:比较了Acidithiobacillus ferrooxidans对环状硫(α-S)和线状硫(μ-S)的利用差异。A. ferrooxidans在这2种单质硫中生长和硫氧化的结果表明该菌对μ-S的利用与α-S明显不同。尽管生长300 h后该菌在2种单质硫基质中达到相同的细胞浓度、相同的pH和硫酸根离子浓度,但在初始阶段细菌细胞在μ-S中生长时的细胞生长速率、pH下降速率和硫酸根增加速率比在α-S中生长时明显快。A. ferrooxidans对这2种硫的初始吸附行为研究表明该细菌对μ-S的平衡吸附量明显高于α-S。SEM、DRIFTS和XRD结果表明,A. ferrooxidans对这2种单质硫表面进行了不同的修饰。选取了11个与硫吸附活化和代谢相关基因通过RT-qPCR进行差异表达分析,结果显示A. ferrooxidans在μ-S中生长时,与疏水性底物转运蛋白和硫代谢相关蛋白表达量比在α-S中生长时明显上调,而吸附和活化相关蛋白表达下调,表明μ-S比α-S易于被A. ferrooxidans适应和活化。
关键词:硫氧化;Acidithiobacillus ferrooxidans;环状硫;线状硫;RT-qPCR
(Edited by Xiang-qun LI)
Foundation item: Projects (50974140, 51274257) supported by the National Natural Science Foundation of China; Project (20090162110054) supported by the Ph.D. Programs Foundation of Ministry of Education of China; Project (2012-18) supported by the Openning Foundation for Precious Instruments of Central South University, China
Corresponding author: Jin-lan XIA; Tel: +86-731-88836944; E-mail: jlxia@csu.edu.cn
DOI: 10.1016/S1003-6326(14)63226-X